- 1The National Engineering Research Center for Crop Molecular Breeding, MARA Key Laboratory of Soybean Biology (Beijing), Institute of Crop Sciences, Chinese Academy of Agricultural Sciences, Beijing, China
- 2Department of Agronomy, Bayero University Kano, Kano, Nigeria
- 3Institute of Industrial Crop Research, Shanxi Academy of Agricultural Sciences, Fenyang, China
Soybean cyst nematode (SCN) has devastating effects on soybean production, making it crucial to identify genes conferring SCN resistance. Here we employed next-generation sequencing-based bulked segregant analysis (BSA) to discover genomic regions, candidate genes, and diagnostic markers for resistance to SCN race 4 (SCN4) in soybean. Phenotypic analysis revealed highly significant differences among the reactions of 145 recombinant inbred lines (RILs) to SCN4. In combination with euclidean distance (ED) and Δsingle-nucleotide polymorphism (SNP)-index analyses, we identified a genomic region on Gm11 (designated as rhg1-paralog) associated with SCN4 resistance. Overexpression and RNA interference analyzes of the two candidate genes identified in this region (GmPLAC8 and GmSNAP11) revealed that only GmSNAP11 significantly contributes to SCN4 resistance. We developed a diagnostic marker for GmSNAP11. Using this marker, together with previously developed markers for SCN-resistant loci, rhg1 and Rhg4, we evaluated the relationship between genotypes and SCN4 resistance in 145 RILs and 30 soybean accessions. The results showed that all the SCN4-resistant lines harbored all the three loci, however, some lines harboring the three loci were still susceptible to SCN4. This suggests that these three loci are necessary for the resistance to SCN4, but they alone cannot confer full resistance. The GmSNAP11 and the diagnostic markers developed could be used in genomic-assisted breeding to develop soybean varieties with increased resistance to SCN4.
Highlights
– We identified rhg1-paralog for the resistance to SCN4.
– GmSNAP11 confers the major resistance of rhg1-paralog to SCN4.
– rhg1, Rhg4, and rhg1-paralog are necessary but cannot confer the full resistance to SCN4.
Introduction
Soybean (Glycine max L. Merrill) is an important legume crop, providing approximately 68% of the world’s supply of protein-rich meals and serving as an excellent source of vegetable oil and renewable fuel. The average yield of soybean increased from 2,183 kg ha–1 in 1994 to 2,769 kg ha–1 in 2019 worldwide, with an annual increase of 23 kg ha–1 (FAOSTAT, 2020). However, biotic and abiotic constraints still limit soybean production (Hartman et al., 2011).
Soybean cyst nematode (SCN, Heterodera glycines Ichinohe) is an economically important pest for soybean causing estimated annual losses of billions of dollars worldwide (Koenning and Wrather, 2010). H. glycines is found in many countries, especially where soybeans are grown on a commercial scale, such as the United States and China (Shannon et al., 2004). The damage caused by SCN is particularly devastating because the aboveground symptoms are not always visible, but stunted roots and reduced nodulation decrease grain yield (Davis and Tylka, 2000; Niblack et al., 2006). Practices such as crop rotation and the use of SCN-resistant soybean varieties are the most effective strategies for SCN control (Davis and Tylka, 2000). In the main soybean production area of China, SCN races 1 (SCN1) and 3 (SCN3) are the predominant races in the Northeast Region while in the Huanghuaihai Region, SCN race 4 (SCN4) is the predominant race (Liu et al., 2019; Shaibu et al., 2020). SCN4 is the most virulent race, however, the genes underlying SCN4 resistance in soybean have not yet been fully identified (Liu et al., 2019).
Numerous SCN-resistant resources have been identified from the abundant soybean germplasm (Li et al., 1991; Arelli et al., 1997; Shaibu et al., 2020). Nevertheless, only a few of these resources have been used in soybean breeding because most of them carry undesirable traits that are difficult to improve using conventional breeding techniques (Li et al., 2014). The use of PI 88788 in SCN resistance breeding programs has led to SCN population shifts, resulting in the emergence of new SCN biotypes (Diers and Arelli, 1999; Vuong et al., 2010).
Soluble N-ethylmaleimide-sensitive factor attachment proteins (SNAPs) have been widely studied in plants and animals. SNAPs are involved in maintaining plasma membrane stability, vesicular trafficking, calcium-binding, cytokinesis, membrane repair, and human genetic diseases including certain cancers (Andrews and Chakrabarti, 2005; Schapire et al., 2008; El Kasmi et al., 2013; Südhof, 2013). In plants, an α-SNAP gene associated with disease resistance is present in most plant genomes (Lakhssassi et al., 2017). In soybean, various loci conferring resistance to SCN have been identified, including rhg1 (Cook et al., 2012) and Rhg4 (Liu et al., 2012). The rhg1 locus contains Glyma.18G022500, which encodes an α-SNAP and is referred to as GmSNAP18 (Liu et al., 2017). The Rhg4 locus contains Glyma.08G108900, which encodes a serine hydroxymethyltransferase (SHMT) and is referred to as GmSHMT08 (Liu et al., 2012). The rhg1 is categorized into rhg1-a and rhg1-b; the rhg1-a acts additively with Rhg4 to confer SCN resistance in Peking-type soybean lines, while GmSNAP18 (rhg1-b) alone confers SCN resistance in PI 88788-type lines (Cook et al., 2012; Liu et al., 2012, 2017). However, both types of resistance require additional genes to confer a wider spectrum of resistance (Liu et al., 2019). For instance, the two major sources (Peking and PI 88788) of SCN resistance are susceptible to SCN4 (Niblack et al., 2006), the predominant race in HuangHuaiHai Region of China (Lian et al., 2017).
The SNAP gene family in soybean contains five members. GmSNAP11 shares a strong similarity with GmSNAP18 and is thought to additively contribute to SCN3 resistance (Li et al., 2016; Lakhssassi et al., 2017; Tian et al., 2019). However, at the time of conducting this research, further molecular evidence for the SCN4 resistance of GmSNAP11 is lacking. Moreover, the resistance function of the SNAP family to SCN4 is rarely reported. Recently, a γ-SNAP gene family was also reported to contribute to SCN resistance (Butler et al., 2021). The resistance of soybean to SCN is highly complex, highlighting the need to identify additional sources of resistance in order to develop new resistant varieties (Liu et al., 2019). The development of such varieties is crucial for the long-term management of SCN (Shaibu et al., 2020).
Functional analysis of soybean genes requires methods for rapid mapping of the genes that control important agronomic traits. A few genes controlling various agronomic traits such as stem growth habit, seed number per pod, hard-seededness, and salt tolerance have been identified by positional cloning (Jeong et al., 2012; Guan et al., 2014; Ping et al., 2014; Sun et al., 2015). Traditional map-based cloning is time-consuming and usually low-throughput. Bulked segregant analysis (BSA) is a simple approach for quickly identifying loci governing traits of interest (Giovannoni et al., 1991; Michelmore et al., 1991). The BSA approach has been used to discover novel genomic regions or genes conferring disease resistance in several legumes (Singh et al., 2016; Pandey et al., 2017; Deokar et al., 2019; Luo et al., 2019).
In the present study, we performed BSA to discover novel genomic regions and identify candidate genes for SCN4 resistance in soybean. Two candidate genes in the rhg1-paralog locus (similar to rhg1), Glyma.11g234400 (GmPLAC8) and Glyma.11g234500 (GmSNAP11), were functionally verified by analyzing genetically transformed hairy roots. In addition to using previously reported kompetitive allele-specific PCR (KASP) markers for rhg1 and Rhg4, we developed a novel diagnostic marker for rhg1-paralog. These markers could be used for the pyramiding of loci for SCN4 resistance in soybean via marker-assisted selection.
Materials and Methods
Plant Materials
Glycine max cv. Jindou 23 (JD23, accession number: ZDD23989) and Huipizhiheidou (HPD, accession number: ZDD02315), with susceptibility and resistance to SCN4, respectively, were crossed, and confirmed F1 plants were self-fertilized to F10 to develop a recombinant inbred line (RIL) population. The HPD landrace was used for gene cloning and vector construction for functional analysis of genes. Two RILs having rhg1+/Rhg4+ background were used for functional analysis of genes. RIL L99, which is susceptible to SCN4, was used for overexpression (OE) analysis; while L9, which is resistant to SCN4, was used for RNA interference (RNAi) analysis. 1025 Chinese soybean accessions were provided by the Research Group of Soybean Genetic Resources (Professor Lijuan Qiu’s lab), Institute of Crop Sciences, Chinese Academy of Agricultural Sciences. Of these accessions, PI 437654, PI 548402 (Peking), PI 90763, PI 88788, PI 209332, and PI 548316 (Cloud) are widely used as indicators to classify SCN HG type and parents to breed varieties with SCN resistance.
Identification of Resistance to Soybean Cyst Nematode 4 (SCN4)
The reaction of the RILs and soybean accessions to SCN4 was determined using female index (FI) values at the Institute of Industrial Crop Research, Shanxi Academy of Agricultural Sciences, China. Mature female soybean cyst nematodes were harvested and the eggs were released as described previously (Arelli et al., 1997). The eggs were hatched at 26°C for a week. Infective Juvenile 2 (J2) nematodes were concentrated by centrifugation at 1000 rpm for 2 min to a final concentration of 1000 J2 mL–1. Each 2-mL aliquot of this inoculum was placed into a 2-cm hole beside the roots of a soybean seedling. Plant roots were individually washed with distilled water 38 days after inoculation. Mature females were counted under a stereomicroscope and the FI on each plant was calculated. Rating of resistance (FI < 10%) and susceptibility (FI > 10%) was used to classify the reactions as described by Schmitt and Shannon (1992) using the following equation:
Construction of Sequencing Libraries and Sequencing
Genomic DNA was isolated from young leaves of the 145 RILs using a genomic DNAsecure plant kit (Tiangen, Beijing, China). The RILs were genotyped for rhg1 and Rhg4. The previously reported KASP markers, GSM381 and GSM383, were used for rhg1 detection (Shi et al., 2015). For Rhg4 genotyping, we developed a modified KASP marker GSM150 based on a previously reported marker GSM191 (Shi et al., 2015), which has the same target SNP but different primer sequences with GSM191.
Based on the analysis of SCN4 resistance, some RILs in the rhg1+Rhg4+ background had different levels of SCN4 resistance. Two bulks were generated from RILs in the rhg1+Rhg4+ background, with 14 RILs each for susceptibility (S-bulk) and resistance (R-bulk) to SCN4. The RILs having FI values within the range of 1.1 to 7.5% were bulked together as R-bulk, while the RILs having FI values within the range of 50.3 to 105.7% were bulked together as S-bulk. Approximately 5 μg DNA samples from the two parental lines and the two bulks were used to construct paired-end sequencing libraries using the TruSeq Nano DNA HT Sample Preparation Kit (Illumina, CA, United States). The sequencing libraries were subjected to sequencing on an Illumina HiSeq 2500 platform to generate 150-bp paired-end reads.
To ensure the reliability of reads, the raw reads were processed through a series of quality control (QC) procedures using in-house C scripts to trim adaptors and remove low-quality reads based on the following criteria: (1) Removing reads with ≥ 10% unidentified nucleotides; (2) Removing reads with > 50% bases having phred quality score ≤ 10; (3) Removing reads with > 10 nucleotides aligned to the adapter, allowing ≤ 10% mismatches; (4) Removing putative PCR duplicates generated by PCR amplification in the library construction process. The clean reads were further rechecked for quality using FASTQC (V0.11.3). The Q30 (99.9% base call accuracy) and the GC content of the clean data were calculated. Consequently, high-quality reads were aligned and mapped to the Glycine max Wm82.a2.v1 reference genome from Phytozome1 using BWA (V0.7.10) with default parameters (Langmead and Salzberg, 2012). The percentage of mapped reads, the average depth, and the coverage ratio were calculated for each of parental lines and bulks. Genome Analysis Toolkit (GATK) HaplotypeCaller function was used to call SNPs and small indels across parental lines and bulks (McKenna et al., 2010). The SNPs were filtered using the GATK Variant Filtration protocol with the following settings: quality by depth (QD) < 4, mapping quality (MQ) < 40, depth of coverage (DP) < 4, MQRankSum < – 12.5.
Identification of Genomic Regions and Candidate Genes for Soybean Cyst Nematode (SCN)
The two bulks were re-sequenced separately, and homozygous SNPs between the parental lines and high-quality SNPs were selected for SNP-index analysis. We used two methods to detect genomic regions associated with SCN resistance using 2,019,213 high-quality SNPs.
Amut is the frequency of the A base in the mutation pool, and Awt is the frequency of the A base in the wild pool; the same is true for Cmut, Gmut, and Tmut.
Single-nucleotide polymorphism (SNP) sites with different genotypes between the two pools were used to calculate the depth of each base in different pools, and the ED value of each locus was calculated as described by a previous study (Hill et al., 2013). The ED values were quintic powered to reduce the effects of noise and increase the effects of large ED values. As a complementary method to reduce the effects of noise, the LOESS fitted curves were calculated using the values of ED5 of all SNPs as described by Hill et al. (2013). The genomic median of all LOESS fitted values plus three standard deviations was set as a correlation threshold to select peak regions (Hill et al., 2013).
Association analysis based on the SNP index is a method used to detect significant differences in genotype frequency between the pools, as indicated by ΔSNP-index, which is calculated as follows:
Maa represents the depth of aa from the maternal parent, Paa represents the depth of aa from the paternal parent, Mab represents the depth of ab from the maternal parent, and Pab represents the depth of ab from the paternal parent.
To reduce the number of false-positive loci, we employed a sliding-window method by calculating the average value of the SNP-index over a 4-Mb window, with an incrementing window of 10 kb (Fekih et al., 2013). The 99th percentiles of all fitted ΔSNP-index values were set as the threshold for identifying genomic regions.
Finally, the ED, SNP-index, and ΔSNP-index values were plotted, and the intersections between candidate regions that were identified using the ED and ΔSNP index methods were designated as final candidate SCN4 resistance-associated regions. We selected SNPs with non-synonymous, splice site acceptor and donor, start and stop codon, and gain and loss within these final candidate regions. Then we selected the SNPs from R-Bulk that was the same as that from HPD and the SNPs from S-Bulk that was the same as that from JD23. Furthermore, we analyzed the ΔSNP-index values of these SNPs and set the theoretical value of a causal SNP to be the third quartile value of their ΔSNP-index values. The regions for which ΔSNP-index values exceeded the threshold were considered candidate regions associated with resistance to SCN4. The identified genes were annotated against Nr, Swiss-Prot, and KOG/COG databases.
Copy Number Analysis
Based on the high-quliaty clean reads, the read number for each base was used to represent its depth. The copy numbers of rhg1 and rhg1-paralog were calculated using the average depth within the 31-kb region of rhg1 and rhg1-paralog, as well as 31 kb upstream and downstream of rhg1 and rhg1-paralog. Specifically, the ratios of the average depth of rhg1 and rhg1-paralog regions to the average depth of their upstream and downstream regions were calculated separately. The copy numbers of rhg1 and rhg1-paralog were estimated as the means of the two ratios compared to their upstream and downstream regions.
Sequencing and Identification of Genetic Variation
The cDNA fragments of the two genes GmPLAC8 (Glyma.11g234400) and GmSNAP11 (Glyma.11g234400) from HPD were cloned and sequenced. Total RNA was isolated from young leaves and roots using TRIZOL kit (TransGen, Beijing, China), and cDNA was synthesized using a FastQuant RT kit (Tiangen, Beijing, China). RNA and cDNA quantities were measured using a NanoDrop spectrophotometer (Thermo Fischer Scientific, Langenselbold, Germany) and the quality of the RNA was checked by 1% agarose gel electrophoresis. PCR amplification was performed using KOD-Plus (Toyobo, Osaka, Japan) with the following cycling parameters: 35 cycles of 94°C 2 min (pre-denaturation), 94°C 15 s (denaturation), 55°C 30 s (annealing), and 68°C 2 min (extension) with 7 min of extension at 68°C. The PCR products were separated on a 1% agarose gel. The primers used for PCR are listed in Supplementary Table 1. The amplified fragments were purified using an EasyPure Quick Gel Extraction kit (TransGen, Osaka, Japan). The gel-purified fragments were cloned using a pEASY Blunt Cloning Kit (TransGen, Beijing, China) and cultured on a solid Luria-Bertani (LB) medium overnight at 37°C. PCR amplification was done to identify positive clones using a Taq kit (TransGen, Beijing, China). The PCR products were separated on a 1% agarose gel to identify positive clones with the correct amplicons. The positive clones were sequenced, and sequences that matched the original HPD sequence of rhg1-paralog were aligned to that from JD23.
The two genes in four SCN-resistant plant introduction lines were also cloned and sequenced, including lines PI 88788 (9 copies), PI 209332 (10 copies), PI 437654 (3 copies), and PI 548402 (3 copies). Total RNA was extracted from the samples using a TRIZOL kit (TransGen, Beijing, China), and cDNA was synthesized using a FastQuant RT kit (Tiangen, Beijing, China). The genes from the plant introduction lines were cloned and sequenced as described for HPD, and the sequences were aligned to HPD sequences to detect genetic variations.
Protein Structure Modeling
The protein structure of GmSNAP11 for cv. HPD and JD23 were generated using SWISS-MODEL (Biozentrum2), and the resulting PDB files were analyzed with VectorNTI-3D Molecular Viewer software (Invitrogen Life Science Software, Frederick, MD).
Functional Analyses of GmPLAC8 and GmSNAP11
Functional analyses of the two SCN-resistant candidate genes identified in soybean (GmPLAC8 and GmSNAP11) were performed using transgenic hairy roots. GmSNAP18 was used as a control in all experiments. Plasmid DNA was purified from positive clones identified in overnight cultures of Escherichia coli in a LB liquid medium using a Tianprep Mini Plasmid Kit (Tiangen, Beijing, China). The pGFPGUSplus (13300 bp) vector (Vickers et al., 2007) was used for OE and RNAi studies. The pGFPGUSplus vector was firstly double digested with the restriction enzymes BglII and BstEII. The coding sequences (CDS) of GmPLAC8, GmSNAP11, and GmSNAP18 were then ligated into pGFPGUSplus to develop OE constructs using In-Fusion. Advantage PCR Cloning Kit (TAKARA, Tokyo, Japan). For the RNAi constructs, the RNAi structures for GmPLAC8, GmSNAP11, and GmSNAP18 were separately ligated into pGFPGUSplus. The primers used to construct the RNAi and OE vectors are listed in Supplementary Table 1.
Hairy Root Transformation
Agrobacterium rhizogenes strain K599 was co-bombarded with OE and RNAi constructs harboring the candidate genes using a Gene Pulser Electroporator (Bio-Rad Hercules, United States). The products were added to a yeast extract peptone (YEP) medium and shaken at 200 rpm at 28°C for one hour. Twenty μL of the product was inoculated onto a solid YEP medium and cultured overnight at 28°C. Positive clones were selected by PCR and stored in glycerol solution at -80°C for further analysis. The resistant (L9) and susceptible (L99) RILs were subjected to hairy root transformation as described previously (Chen et al., 2018). Transgenic hairy roots were identified by detecting green fluorescent protein with a LUYOR-3260 flashlight (LUYOR, Shanghai, China). Positive hairy roots were propagated twice and used for the SCN inoculation experiments.
Nematode Infection of Transgenic Hairy Roots
Infective second-stage juveniles (J2) were used for this experiment. Nematodes were surface sterilized with sterilizing solution [0.004% (w/v) mercuric chloride, 0.004% (w/v) sodium azide, and 0.002% (v/v) Triton X-100] for 8 min followed by five washes with sterile water and resuspended in 0.1% (w/v) agarose. Hairy roots (3–4 cm) grown on ICM were inoculated approximately 1 cm above the root tip with 200 ± 25 J2s per root in a 25 mL volume. The plates were incubated in the dark for 30 days at room temperature. The experiment was conducted independently three times, and the number of cysts was counted under a stereomicroscope.
RNA Isolation and qPCR
Total RNA was isolated from root tissues using a TRIZOL kit (TransGen, Beijing, China), and cDNA was synthesized using a FastQuant RT kit (Tiangen, Beijing, China). Quantitative RT-PCR was carried out using an Applied Biosystems QuantStudio 7 Flex Real-time PCR system (Applied Biosystems, CA, United States). Gene-specific primer pairs (Supplementary Table 1) were used, and all quantitative RT-PCR assays were carried out using three biological and three technical replicates. The soybean Actin gene was used as an internal control. PCR was performed using the following cycling parameters: 95°C for 3 min, 60°C for 30 s, 40 cycles of 95°C for 15 s, and 60°C for 1 min.
SNP Marker Development and Validation of Candidate Genomic Regions
The KASP marker GSM151 for rhg1-paralog was designed based on the splice donor SNP in GmSNAP11 (Supplementary Figure 1) and validated by Sanger sequencing of amplification products between cv. HPD and JD23. The KASP assay was also performed for rhg1 and Rhg4 loci to analyze the additive effects of these three loci. The KASP primers used in this study are shown in Supplementary Table 2. The KASP reaction mixture was prepared using the protocol described by LGC Genomics3. The following cycling conditions were used: 15 min at 95°C, followed by 10 touchdown cycles of 20 s at 94°C, 1 min at 65–57°C (dropping 0.8°C per cycle), 30 cycles of 20 s at 94°C, and 1 min at 57°C. The fluorescence levels were detected using a PHERAstarplus SNP Microplate Reader (BMG Labtech, Offenburg, Germany), imported using Kraken software by following the manufacturer’s guidelines, and analyzed using KLUSTERCALLER software (version 3.4.1.36; LGC Hoddesdon, United Kingdom). The KASP assay was firstly performed on 30 soybean accessions and 145 RILs, and then on 1025 Chinese soybean accessions. The 1025 soybean accessions and their origin were previously described in detail (Abdelghany et al., 2020). The geographical distribution of accessions with three Rhg loci (rhg1, Rhg4, and rhg1-paralog) in China was constructed with ArcGIS v10.0 (ESRI, Redlands, CA, United States).
Results
Phenotypic Variation and Construction of Extreme Bulks for Soybean Cyst Nematode 4 (SCN4) Resistance
The SCN4-resistance of a population of 145 recombinant inbred lines (RILs, F2:10) derived from a cross between soybean cv. Jindou23 (JD23, susceptible to SCN4) and Huipizhiheidou (HPD, resistant to SCN4) were evaluated. The distribution of the female index (FI) among the RIL populations was observed to follow a normal distribution (Supplementary Figure 2A), and highly significant differences (P < 0.0001) were observed in reactions to SCN4 among the RILs (Supplementary Figure 2B).
The genotypes of rhg1 and Rhg4 in the 145 RILs were determined using previously reported KASP markers, GSM381 and GSM383 (for rhg1), and a modified KASP marker GSM150 (for Rhg4), The RILs with the rhg1+Rhg4+ genotype exhibited various reactions to SCN4, ranging from resistant to susceptible (Supplementary Table 3), suggesting that additional alleles exist for this trait. Therefore, we selected 14 resistant and 14 susceptible lines with the rhg1+Rhg4+ genotype to prepare the resistant bulk (R-Bulk) and susceptible bulk (S-Bulk). The FI value ranged from 1.1 to 7.5% among the 14 resistant lines, and 50.3 to 105.7% among the 14 susceptible lines.
Whole-Genome Resequencing, Reads Mapping, and Identification of SNPs
Whole-genome resequencing of the bulks was conducted and 183.37 Gb of reads were obtained. After filtering, 159.37 Gb of clean reads were obtained, including 43.91 Gb of clean reads for parental line JD23, 36.94 Gb for the S-Bulk, 46.87 Gb for parental line HPD, and 31.65 Gb for the R-Bulk with their Q30 values ≥ 88.44% (Supplementary Table 4). Over 98.6% of the clean reads could be mapped to the reference genome (w82.a2.v1). The average sequencing depth was 27 × and 32 × for the resistant and susceptible bulks, respectively, and 40 × and 41 × for parental line JD23 and HPD, respectively. More than 98% of the genome had at least 1 × coverage in all four samples (Supplementary Table 5). SNPs between the parental lines and the Williams 82 reference genome (W82.a2v1) were called and putative variations between the parental lines were identified by selecting SNPs that were unique to a single parent. Ultimately, 2,019,213 high-quality SNPs were identified between parental lines.
Identification of Candidate Genomic Regions for Soybean Cyst Nematode 4 (SCN4) Resistance
Euclidean distance (ED) analysis was performed using SNPs with different genotypes between the two bulks. Based on the threshold of 0.16, genomic regions on chromosomes 10, 11, and 17 were identified as important loci for SCN4 resistance, with a total genomic size of 24.31 Mb (Figure 1 and Supplementary Figure 3). A total of 2008 genes are included in these genomic regions (Supplementary Table 6).
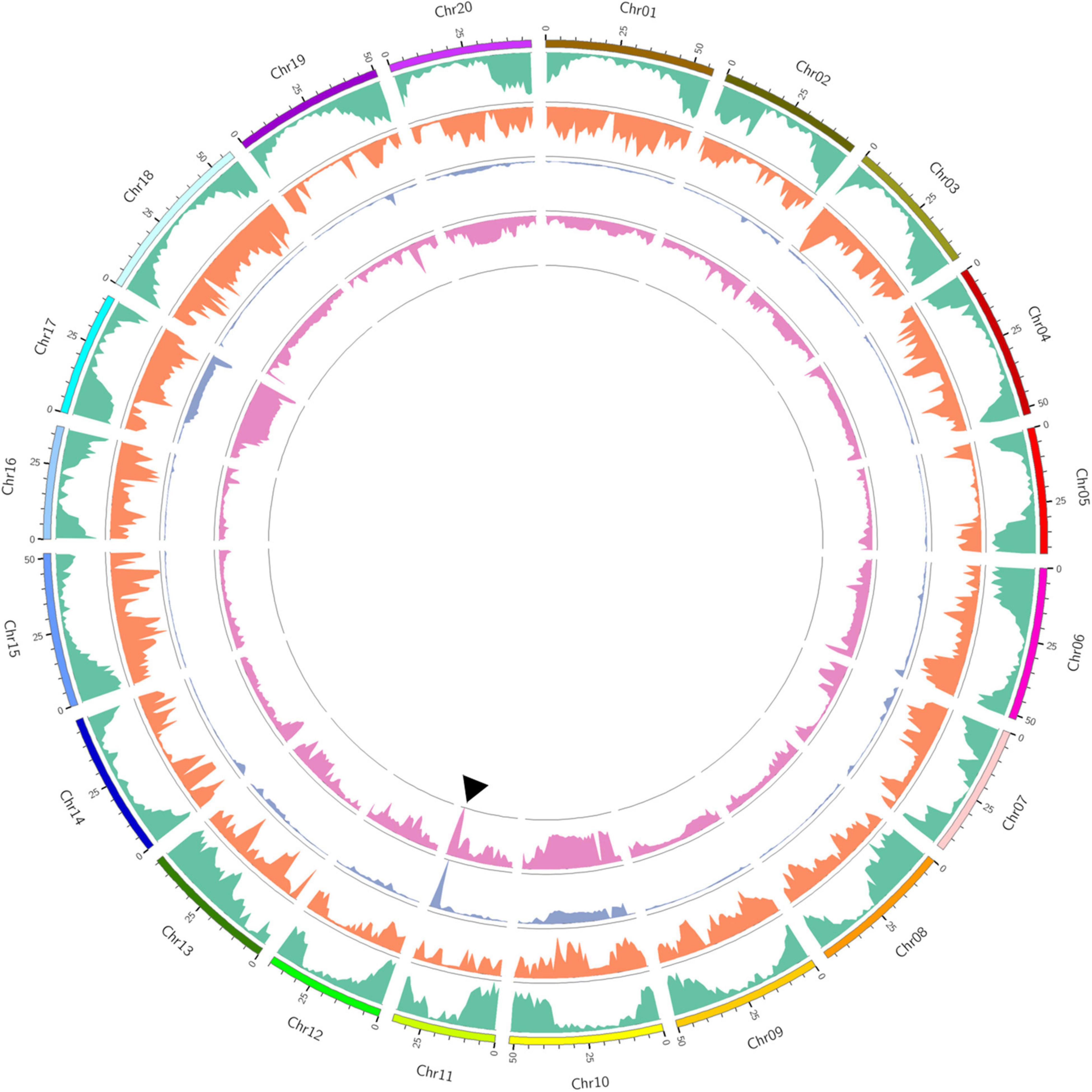
Figure 1. Bulked segregant analysis (BSA)-seq analysis of the recombinant inbred line (RIL) population. Outer to inner circles: chromosome distribution, gene distribution (green color), single-nucleotide polymorphism (SNP) density distribution (orange color), euclidean distance (ED) value distribution (cyan color), and ΔSNP-index distribution (rose color). The solid triangle indicates the peak position of the overlapping genomic regions at Gm11 using both ED and ΔSNP-index methods.
Also, ΔSNP-index analysis was conducted to identify genomic regions associated with SCN4 resistance (Figure 1 and Supplementary Figure 4). According to the threshold value of 0.31, genomic regions on chromosome 07, 11, and 14 were identified for resistance to SCN4, with a total genomic size of 9.42 Mb. A total of 1452 genes were included in these genomic regions (Supplementary Table 7). By comparing the ED and ΔSNP-index results, an overlapping genomic region spanning 5.08 Mb on chromosome 11 was found to be associated with SCN4 resistance (Supplementary Table 8).
Candidate Genes for Soybean Cyst Nematode 4 (SCN4) Resistance
Annotations of the genes identified in the genomic region on chromosome 11 were performed. In this region, we identified 8429 SNPs with different genotypes between the two parents. Most of these SNPs mapped to non-coding regions, such as intergenic regions, upstream and downstream regions, and introns of the annotated genes. However, we also identified 214 synonymous SNPs, 237 non-synonymous SNPs, 31 SNPs affecting splice sites, and 28 SNPs affecting start or stop codons in the coding regions of the genes (Supplementary Table 9). The non-synonymous SNPs and the SNPs affecting splice sites and start or stop codons were subjected to additional screening in which SNPs with different genotypes in the resistant parent and R-Bulk were filtered out. Ultimately, 46 non-synonymous SNPs, nine SNPs affecting splice sites, and seven SNPs affecting start codons were identified. We re-evaluated the ΔSNP-index values of these 62 SNPs and selected 15 SNPs with values higher than the third quartile value (0.692) due to their closeness to the theoretical value of a causal SNP (ΔSNP-index = 1).
The 15 SNPs were located in 12 annotated genes (Table 1). Surprisingly, of the 12 genes, two genes (Glyma.11g234400 and Glyma.11g234500) had high similarity to the previously identified SCN resistance genes Glyma.18G022600 and Glyma.18G022500 at rhg1. Glyma.11g234400 encodes (Z)-gamma-bisabolene synthase 1-related protein (a homolog of PLAC8 family protein) and Glyma.11g234500 encodes an α-SNAP, therefore, we designated these two genes as GmPLAC8 and GmSNAP11, respectively. The genomic region harboring GmPLAC8 and GmSNAP11 shows high collinearity with rhg1, the major SCN resistance locus; therefore, this region was designated as rhg1-paralog. We focused our analysis on the rhg1-paralog region and the two candidate genes.
Copy Number Variation in rhg1-Paralog
We examined the copy number of rhg1-paralog in various lines. No copy number variation within the 31-kb rhg1-paralog region was observed. There was only one copy of the 31-kb region of rhg1-paralog of JD23, HPD, R-Bulk, and S-Bulk (Supplementary Figure 5A), whereas there were three copies of rhg1 on chromosome 18 in HPD, R-Bulk, and S-Bulk (Supplementary Figure 5B).
Sequencing and Identification of GmPLAC8 and GmSNAP11 Genetic Variants
To identify the sequence variation between the resistant and susceptible parents, we isolated and cloned the cDNA sequences of the two newly identified genes from parental line HPD and aligned them with the sequences found in parental line JD23. For GmPLAC8, four SNPs and a 93-base pair (bp) deletion were detected in HPD (Supplementary Figure 6), while for GmSNAP11, three SNPs and a 17-bp insertion were detected in HPD (Supplementary Figure 7). Specifically, for GmPLAC8, the A/G SNP at position 1083 and the T/C SNP at position 1530 are synonymous, whereas the A/T SNP at position 886 and the G/A SNP at position 959 lead to Ala/Thr and Arg/Gln transitions at amino acid positions 296 and 320, respectively. The 93-bp deletion causes a 31-amino acid deletion (amino acids 368–398) in HPD. For GmSNAP11, the T/G SNP at position 108 and the G/A SNP at position 681 are synonymous, whereas the G/A SNP at position 535 causes an Ala/Thr transition at amino acid 179. The 17-bp insertion in HPD causes a frameshift mutation, generating a truncated GmSNAP11 protein (Supplementary Figure 8).
Alignment analysis between cDNA and genomic DNA of GmSNAP11 from JD23 and HPD indicated that the insertion could be attributed to the above-mentioned SNP (Gm11: 32969916) discovered by BSA-sequencing. A G/T transition of this site causes an incorrect splice. As a result, a 17-bp fragment of the seventh intron in JD23 is retained in the GmSNAP11 transcript for HPD, ultimately resulting in a frame-shift mutation. We cloned and sequenced these two genes in several resistant and susceptible varieties. For GmSNAP11, we detected similar sequences in four resistant plant introduction lines sequenced (Supplementary Figure 9). The 3D protein structure of GmSNAP11 showed significant variation between JD23 and HPD (Supplementary Figure 10).
GmSNAP11 Contributes to Soybean Cyst Nematode 4 (SCN4) Resistance
To functionally validate the two candidate genes, we performed hairy root transformation of two RILs (L9 and L99) with the same rhg1+Rhg4+ backgrounds but different genotypes for GmSNAP11 and GmPLAC8 to alter the expression of the two genes (and GmSNAP18 as a control). L9 (rhg1+, Rhg4+, and rhg1-paralog+) shows strong resistance to SCN4 (FI = 5.4), whereas L99 (rhg1+, Rhg4+, and rhg1-paralog–) was susceptible to SCN4 (FI = 66.5). Gene expression analysis indicated that the expressions of GmPLAC8, GmSNAP11, and GmSNAP18 were partially suppressed by RNAi in transformed L9 hairy roots (Figure 2A). Highly significant differences (P < 0.0001) in the number of cysts were detected between L9 hairy roots transformed with different RNAi constructs (Figure 2C). The number of cysts was significantly higher when GmSNAP11 expression was suppressed in the resistant line (Figure 2C). The highest number of cysts was observed in L9 hairy roots with suppressed GmSNAP18 expression. By contrast, L9 hairy roots with partially suppressed GmPLAC8 expression showed no significant difference in the average cyst number compared to the control (Figure 2C).
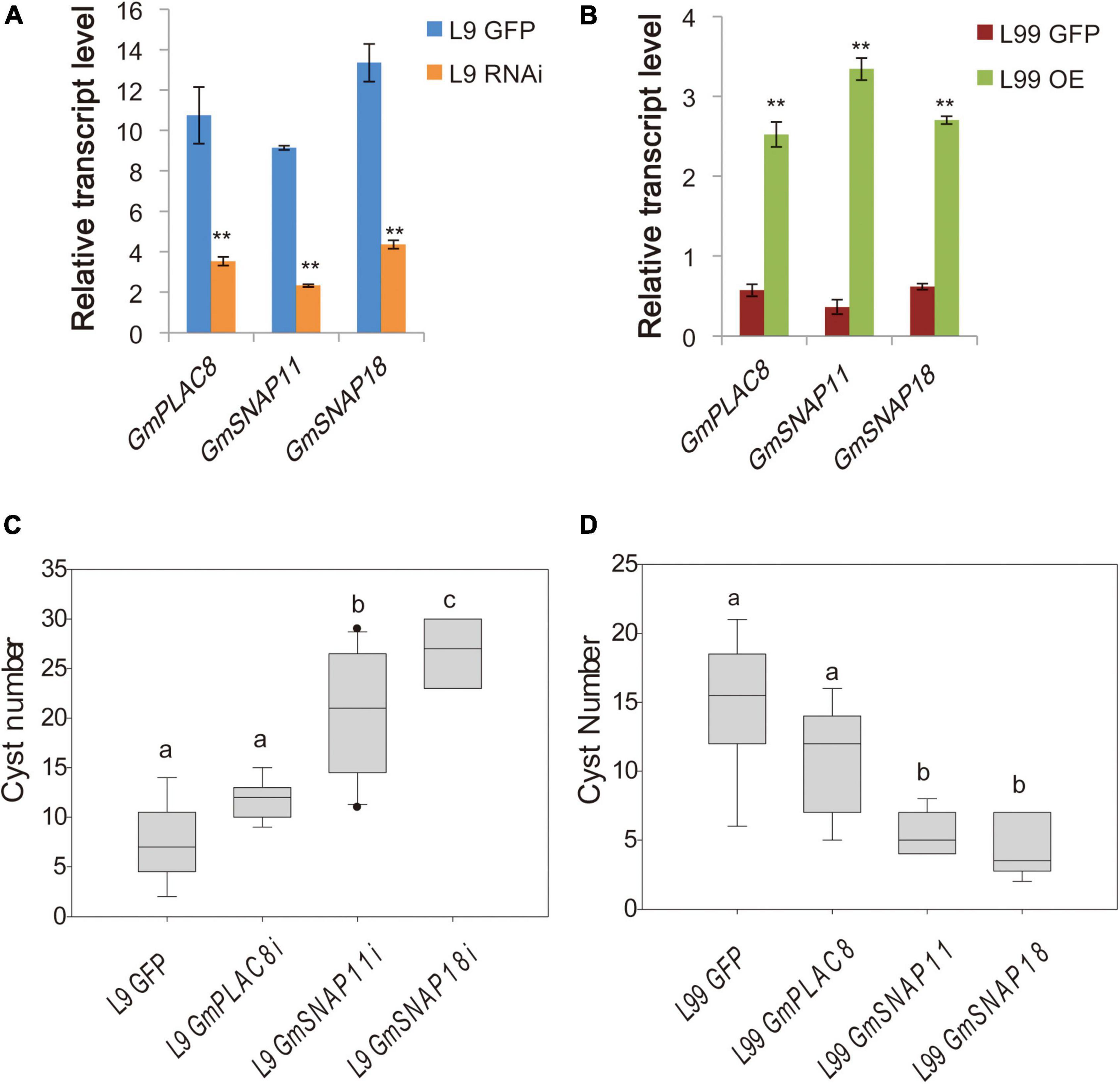
Figure 2. Relative transcript levels and cyst counts in various lines. (A) Relative transcript levels in the RNAi and (B) overexpression lines. The mean and SE of nine samples are shown. ** represents a significant difference at P < 0.01. (C) Cyst counts in soybean cyst nematode 4 (SCN4)-resistant RIL L9 (rhg1+, Rhg4+, rhg1-paralog+) with suppressed expression (RNAi) of these genes and (D) SCN4-susceptible RIL L99 (rhg1+, Rhg4+, rhg1-paralog–) overexpressing these genes. The different letters above the boxes represent different significant levels at P < 0.05.
The overexpression of the candidate genes and GmSNAP18 in the L99 (susceptible line) hairy roots showed that the expression levels are approximately 10-fold higher than that in the control (Figure 2B). The number of cysts significantly differed (P < 0.0001) among hairy roots transformed with different overexpression constructs (Figure 2D). Transgenic hairy roots overexpressing GmSNAP18 had the fewest cysts (4.3), which was not significantly different from the number of cysts recorded in hairy roots overexpressing GmSNAP11 (5.4) (Figure 2D). By contrast, the number of cysts in hairy roots overexpressing GmPLAC8 was not significantly different (P > 0.05) from that of the control.
The Additive Effects of Three Loci on Soybean Cyst Nematode 4 (SCN4) Resistance
To assess the additive effects of the three resistance loci (rhg1, Rhg4, and rhg1-paralog), we developed a diagnostic KASP marker for GmSNAP11 (GSM151) that could successfully identify the SNP affecting the splice site (Supplementary Figure 11). This KASP marker clearly differentiated between the resistant and susceptible lines as well as their respective bulks. We tested this diagnostic marker, together with previously reported KASP markers for rhg1 and Rhg4, by evaluating 30 soybean accessions and the 145 RILs for rhg1 (GmSNAP18, Supplementary Figures 11A,B), Rhg4 (GmSHMT08, Supplementary Figure 11C), and rhg1-paralog (GmSNAP11, Supplementary Figure 11D). All SCN4-resistant accessions harbored three resistance loci, while some accessions (e.g., Peking, PI 90763 and PI 84751) with three resistance loci were still susceptible to SCN4 (Table 2). Other accessions harborining one, two, or none of the resistant Rhg loci were susceptible to SCN4.
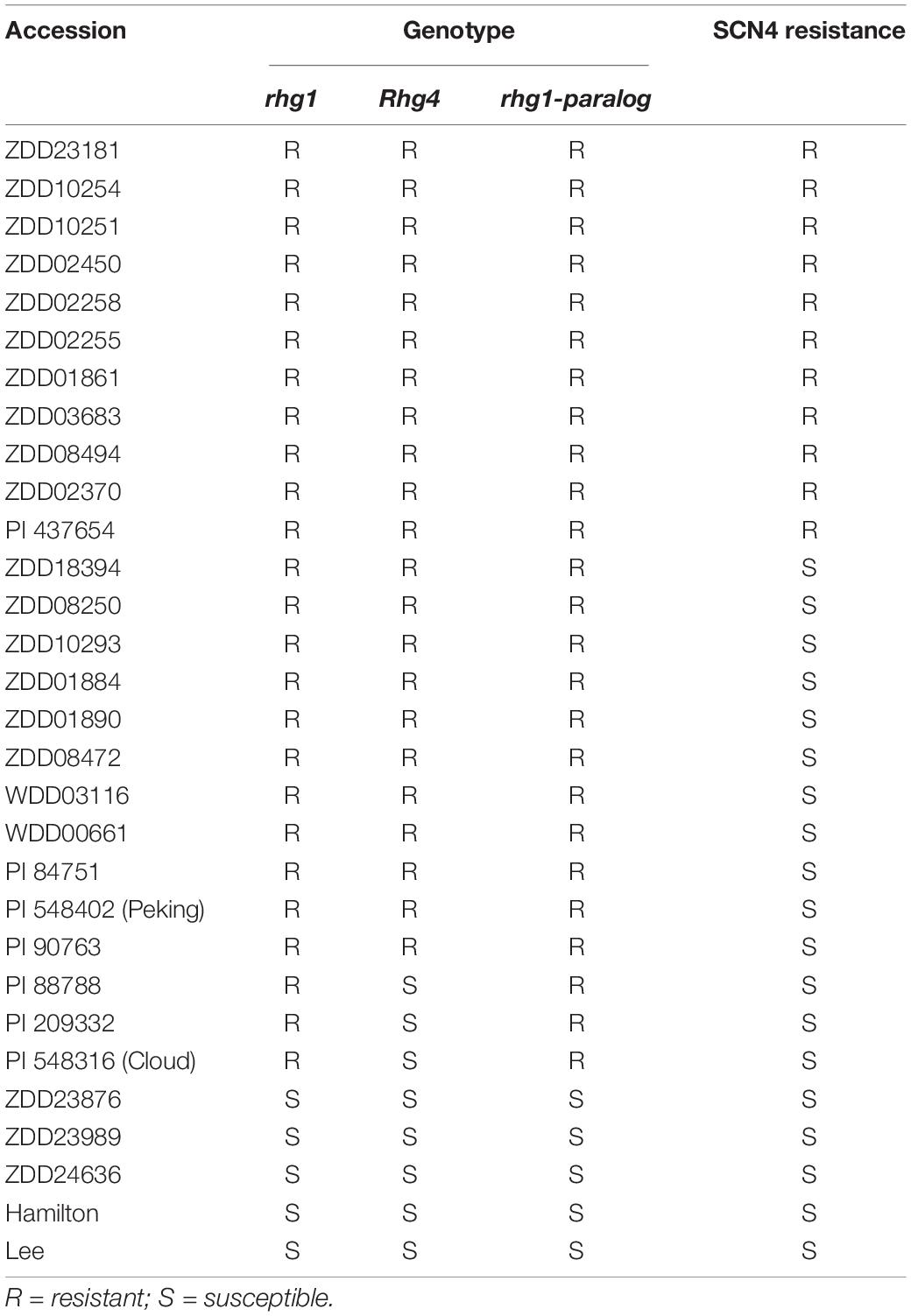
Table 2. Genotypes of three loci for soybean cyst nematode 4 (SCN4) resistance in 30 soybean accessions.
Among the 145 RILs, lines harboring rhg1, Rhg4, and rhg1-paralog showed significantly higher resistance to SCN4 than the other lines, although a wide range of resistance was observed among them. Whereas, no significant differences were observed among lines harboring one, two, or none of the resistance loci (Figure 3). Specifically, all 14 SCN4-resistant lines (FI ranging from 1.13–7.53) harbor rhg1, Rhg4, and rhg1-paralog, while 12 lines with all three genotypes were still susceptible to SCN4, with FI values ranging from 28.27–105.73 (Supplementary Table 3). Lines harboring only rhg1 and Rhg4 had FI ranging from 48.57–86.63, and lines harboring only rhg1 and rhg1-paralog had FI ranging from 44.49–104.70. Lines with only the rhg1-paralog locus had FI ranging from 34.622–118.23. Lines lacking all three resistance loci had FI ranging from 58.6–156.77.
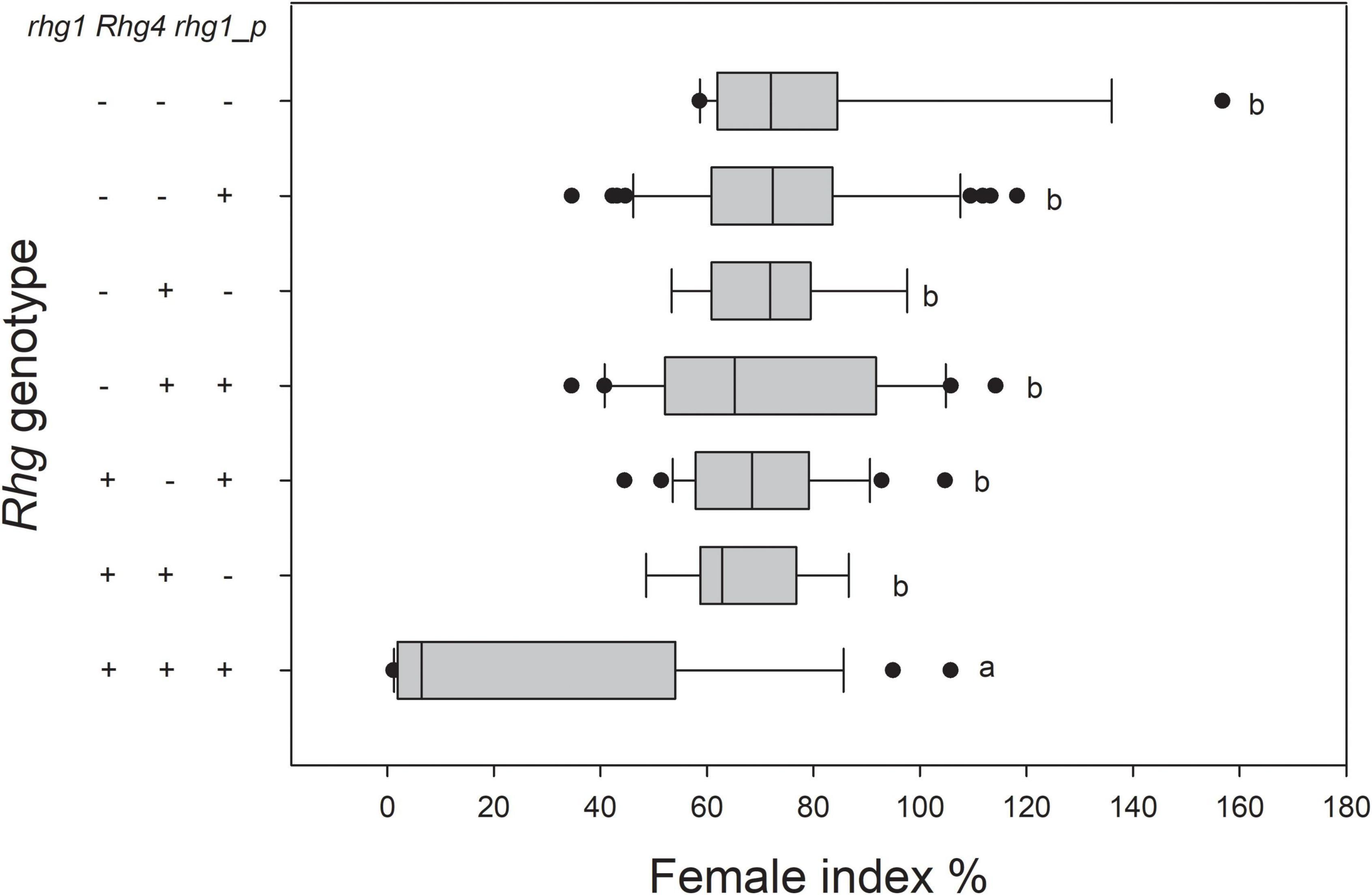
Figure 3. Reaction of RILs to SCN4 based on pyramiding of the rhg1, Rhg4, and rhg1-paralog loci (+ = present; - = absent). There were significant differences between the Rhg genotypes at P < 0.05. rhg1-p represents rhg1-paralog.
Distributions of the Three Rhg Loci in China
Finally, we examined the genotypes of the three resistance loci in 1025 Chinese accessions to explore their distribution in China. For rhg1, rhg1b is distributed across three major ecoregions in China, while rhg1a is primarily distributed across the Huanghuaihai Region of China (Figure 4A). The distribution of Rhg4 is similar to that of rhg1a (Figure 4B), whereas rhg1-paralog shows a wide distribution across China (Figure 4C). Since SCN4 resistance at least relies on rhg1, Rhg4, and rhg1-paralog, we also mapped the distribution of the genotypes of accessions with all three resistance loci. As shown in Figure 4D, accessions with all three resistance loci are primarily distributed across the Huanghuaihai Region of China, where SCN4 is the predominant race of SCN. Additionally, these accessions with three loci were also evaluated for SCN4 resistance. As expected, 61% of these accessions exhibited resistance to SCN4, while 39% showed susceptible phenotype (Supplementary Table 10).
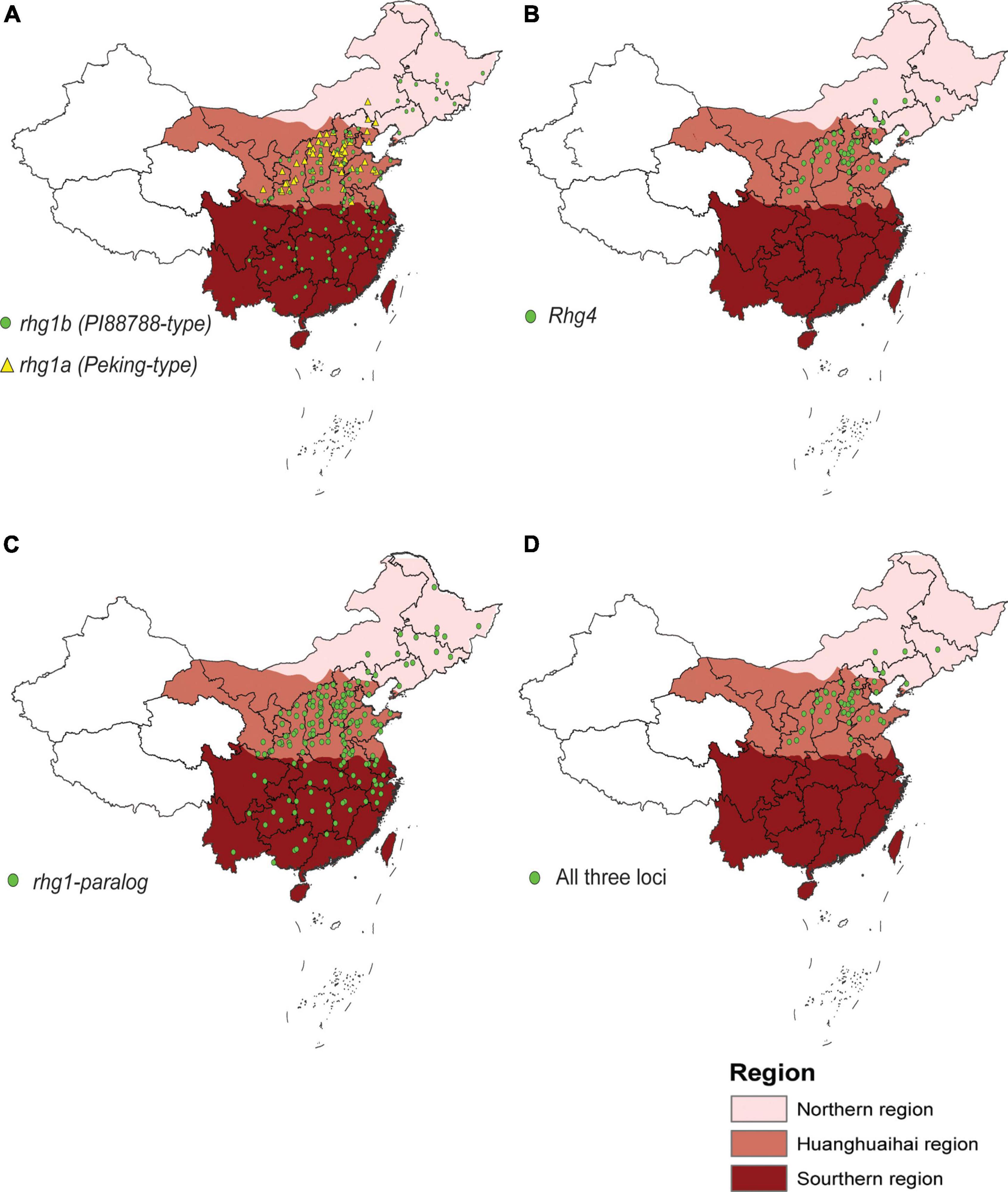
Figure 4. Map showing the distribution of resistance loci in 1025 Chinese accessions across Northern, Huanghuaihai and Southern regions of China. (A) rhg1a and rhg1b; (B) Rhg4; (C) rhg1-paralog; and (D) all three Rhg loci.
Discussion
The damage caused by SCN is devastating and an established SCN population is difficult to eradicate. The two Rhg loci (rhg1 and Rhg4) for SCN act additively to confer resistance, but this resistance is not sufficient. Another minor gene, GmSNAP11, has been assumed to have an additive effect with GmSNAP18 in mediating SCN3 resistance (Li et al., 2016; Lakhssassi et al., 2017). However, SCN4 is the most virulent race, causing significant yield losses annually. The genes conferring resistance to SCN4 in soybean have not yet been fully identified or mapped (Liu et al., 2019).
Over the past few decades, map-based cloning is the most common approach for identifying and isolating candidate genes in many crops. The effectiveness of conventional mapping is limited because it is time-consuming, labor-intensive, and costly to develop marker sets (Lindner et al., 2012). Few genes have been functionally analyzed in soybean compared to other model species (Xia et al., 2013). In the present study, we successfully employed next-generation sequencing-based BSA to identify new genomic regions governing SCN4 resistance. Using this approach, we quickly associated loci with candidate genomic regions, greatly reducing the workload and saving time. We were also able to detect many SNPs and small indels between the parental lines and bulks. Indeed, causal mutations of candidate genes can be identified by detailed analysis of SNPs and indels in candidate genomic regions after fine mapping.
Resequencing of the R- and S-bulks revealed a causal region on chromosome 11 with a physical size of 5.08 Mb, which is very large, even with an average genome coverage of > 35 × for the two parental lines and bulks. However, similar coverage has been reported in soybean (Campbell et al., 2016; Dobbels et al., 2017; Song et al., 2017). Copy number variation is known to contribute to rhg1-mediated resistance to SCN (Cook et al., 2012). In the current study, copy number analysis showed that there was no copy number variation, but a splice donor SNP was identified for GmSNAP11. Therefore, we speculate that the SNP of rhg1-paralog rather than copy number variation contributes to SCN4 resistance. The structural variation in the 3D protein structure of GmSNAP11 between JD23 and HPD points to a clear difference in this protein between resistant and susceptible lines. Indeed, several studies have detected SNPs for GmSNAP11 and suggested that this gene might function in SCN3 resistance by contributing additively to resistance with GmSNAP18 (Li et al., 2016; Lakhssassi et al., 2017; Tian et al., 2019).
From the validation experiment using hairy root transformation, GmPLAC8 did not appear to contribute to SCN4 resistance. Similar results have been previously reported for SCN3 resistance (Cook et al., 2014; Li et al., 2016). For GmSNAP11, however, the number of cysts in hairy roots increased significantly when the expression of this gene was suppressed. Also, overexpressing resistance-type GmSNAP11 in susceptible lines significantly reduced the number of cysts compared to the control. GmSNAP11 may function additively with GmSNAP18 to mediate SCN3 resistance, as determined in previous studies (Lakhssassi et al., 2017; Tian et al., 2019). Specifically, the presence of the GmSNAP11 resistance allele combined with GmSNAP18 decreased the FI from 12.59% in lines having only GmSNAP18 to 4.48% for SCN3 (Lakhssassi et al., 2017). Our study suggests GmSNAP11 also confers the resistance to SCN4 in the presence of rhg1 and Rhg4. Moreover, no significant difference between GmSNAP18 and GmSNAP11 cyst counts was observed for overexpressed transgenic lines. Both of them exhibited increased resistance to SCN4 when overexpressed constitutively even in the presence of rhg1, suggesting the abundance of resistant SNAPs (SNAP18 and SNAP11) may play the most important role for the resistance to SCN4. Since GmSNAP11 presents a high sequence similarity with GmSNAP18, the CRISPR/Cas9-based gene editing will be employed in our subsequent study to exactly distinguish the effects between SNAP11 and SNAP18 on the resistance to SCN4.
It has been reported that the GmSNAP18 and GmSNAP11 are more highly related to each other than the other GmSNAP family members. Both genes usually cluster together in phylogenetic analysis, suggesting they are closely related (Lakhssassi et al., 2017). However, GmSNAP18 is more closely related to the ancestral SNAP genes than GmSNAP11. Among GmSNAP genes, GmSNAP11 and GmSNAP18 are highly expressed in most tissues (Lakhssassi et al., 2017).
The α-SNAPs encoded by rhg1 are unusual α-SNAP proteins that bind less well to wild-type N-ethylmaleimide-sensitive factor and can disrupt vesicle trafficking, eventually leading to cell death (Bayless et al., 2016). As the host syncytium develops, the relative abundance of rhg1-encoded defective α-SNAP variants strongly increases, which disrupts syncytium viability and restricts nematode growth and reproduction (Bayless et al., 2016). Furthermore, the α-SNAPs encoded by GmSNAP18 and GmSNAP11 are major sources of total α-SNAP proteins in soybean (Bayless et al., 2018).
Taking our observations together, considering the similar features of GmSNAP11 and GmSNAP18, we propose that the mechanism by which GmSNAP11 confers resistance to SCN is similar to that employed by GmSNAP18. We demonstrated that overexpressing GmSNAP (GmSNAP11 and GmSNAP18) in the susceptible RIL (rhg1+Rhg4+rhg1-paralog–) reduced the cyst number (elevated resistance) when inoculated with SCN4. Therefore, we suggest that, like GmSNAP18, GmSNAP11 confers resistance via the accumulation of impaired α-SNAP. Under SCN infestation, GmSNAP18 and GmSNAP11 are more highly expressed in most tissues and GmSNAP18 is the most highly upregulated gene, followed by GmSNAP11 (Lakhssassi et al., 2017). It is therefore expected that cultivars harboring both GmSNAP18 and GmSNAP11 would exhibit elevated resistance because their additive effect would lead to increased accumulation of impaired α-SNAP.
In the present study, we successfully transformed a SNP into a KASP marker and used it, along with two previously developed KASP markers, to examine the additive effects of GmSNAP11, GmSNAP18, and GmSHMT08 on SCN4 resistance. The pyramiding effect of these genes on SCN4 seems more complicated than that on SCN3. Tian et al. (2019) showed that pyramiding these three loci conferred more resistance to SCN3 compared to lines containing only GmSNAP18 and GmSHMT08. However, in this study, all SCN4-resistant RILs harbored all three genes, whereas several lines harboring the three genes were still susceptible to SCN4, suggesting that these three genes are necessary for the resistance to SCN4 but these genes alone cannot confer full resistance. Indeed, several studies have demonstrated that SCN resistance in soybean is a very complicated trait (Bayless et al., 2016, 2018; Lakhssassi et al., 2017; Liu et al., 2019; Patil et al., 2019). Several other genes might also contribute to the resistance to SCN3 (Tran et al., 2019) and SCN4 (Liu et al., 2019). The presence of other genes contributing to SCN resistance might explain why we observed variations in cyst number among the soybean accessions and RILs despite having similar pyramiding of the three Rhg loci. Nonetheless, the rhg1, Rhg4, and rhg1-paralog loci are major contributors to SCN resistance and our analysis highlighted the importance of GmSNAP11 in contributing additively to SCN4 resistance. In addition, we demonstrated the potential of using these KASP markers to mine resistance alleles for SCN4 in breeding programs.
Analysis of the distribution of accessions with all three resistance loci suggested that the resistant genotypes originated from the Huanghuaihai Region of China. In the soybean production area of this region, SCN4 is the predominant race of SCN, but the genes conferring specific resistance to SCN4 have not yet been identified (Liu et al., 2019). The Huanghuaihai Region is seriously affected by SCN4, likely explaining why genotypes harboring the three major Rhg resistance loci evolved in this region.
Conclusion
We successfully used next-generation sequencing-based BSA to identify novel genomic regions on chromosome 11 (designated as rhg1-paralog) for SCN4 resistance in soybean. Of the two genes identified, GmSNAP11 was successfully validated as a gene that contributes additively to SCN4 resistance. GmSNAP18 alone cannot confer sufficient resistance to SCN4, as additional genes acting singly or in combination with GmSNAP18 are needed to enhance SCN4 resistance in soybean. GmSNAP11 confers resistance to SCN4 via the accumulation of impaired SNAP, which is similar to the mode-of-action of GmSNAP18. Therefore, the α-SNAP gene family confers SCN resistance through the accumulation of impaired α-SNAP. The diagnostic marker developed for GmSNAP11 in the current study and the existing markers for rhg1 and Rhg4 could be used for marker-assisted selection to identify germplasm with resistance at these loci and to enhance future breeding programs aimed at developing soybean varieties with strong resistance to SCN4.
Data Availability Statement
The original contributions presented in the study are publicly available. This data can be found here: https://ngdc.cncb.ac.cn/, GVM000330.
Author Contributions
BL and JS conceived, designed, and supervised the experiments. JM developed the RIL population and conducted field phenotyping. BL performed the BSA experiment. AS performed gene cloning, functional validation of the identified genes, performed the KASP experiment, interpreted the results, and prepared the draft manuscript. YF and YH assisted in vector construction and hairy root transformation. YF, AA, and MA extracted DNA from the accessions used for the KASP experiment. HH assisted with SCN inoculation and the KASP experiment. BL, JS, and SZ contributed to the improvement and final editing of the manuscript. All authors read and approved the final manuscript.
Funding
This research was supported by National Natural Science Foundation of China (Nos. 32161143033, 32001574, and 31301345) and Agricultural Science and Technology Innovation Program of Chinese Academy of Agricultural Sciences.
Conflict of Interest
The authors declare that the research was conducted in the absence of any commercial or financial relationships that could be construed as a potential conflict of interest.
Publisher’s Note
All claims expressed in this article are solely those of the authors and do not necessarily represent those of their affiliated organizations, or those of the publisher, the editors and the reviewers. Any product that may be evaluated in this article, or claim that may be made by its manufacturer, is not guaranteed or endorsed by the publisher.
Acknowledgments
We thank Xueyi Liu (Institute of Industrial Crop Research, Shanxi Academy of Agricultural Sciences, China) for providing the RIL population and determining the resistance to SCN4, Lijuan Qiu (Institute of Crop Sciences, Chinese Academy of Agricultural Sciences) for providing Chinese soybean accessions, and Yuxi Duan (Shenyang Agricultural University) for technical support on the manipulation of SCN.
Supplementary Material
The Supplementary Material for this article can be found online at: https://www.frontiersin.org/articles/10.3389/fpls.2022.939763/full#supplementary-material
Footnotes
- ^ https://phytozome.jgi.doe.gov/pz/portal.html
- ^ https://swissmodel.expasy.org/
- ^ www.lgcgroup.com/genomics
References
Abdelghany, A. M., Zhang, S., Azam, M., Shaibu, A. S., Feng, Y., Li, Y., et al. (2020). Profiling of seed fatty acid composition in 1025 Chinese soybean accessions from diverse ecoregions. Crop J. 8, 635–44. doi: 10.1016/j.cj.2019.11.002
Andrews, N. W., and Chakrabarti, S. (2005). There’s more to life than neurotransmission: the regulation of exocytosis by synaptotagmin VII. Trends Cell Biol. 15, 626–31. doi: 10.1016/j.tcb.2005.09.001
Arelli, A., Myers, O., Gibson, P., and Wilcox, J. (1997). Soybean germplasm resistant to races 1 and 2 of Heterodera glycines. Crop Sci. 37, 1367–9. doi: 10.2135/cropsci1997.0011183X003700040055x
Bayless, A. M., Smith, J. M., Song, J., McMinn, P. H., Teillet, A., August, B. K., et al. (2016). Disease resistance through impairment of α-SNAP–NSF interaction and vesicular trafficking by soybean Rhg1. Proc. Natl. Acad. Sci. U S A. 113, 7375–82. doi: 10.1073/pnas.1610150113
Bayless, A. M., Zapotocny, R. W., Grunwald, D. J., Amundson, K. K., Diers, B. W., and Bent, A. F. (2018). An atypical N-ethylmaleimide sensitive factor enables the viability of nematode-resistant Rhg1 soybeans. Proc. Natl. Acad. Sci. U S A. 115, 4512–21. doi: 10.1073/pnas.1717070115
Butler, K. J., Fliege, C., Zapotocny, R., Diers, B., Hudson, M., and Bent, A. F. (2021). Soybean cyst nematode resistance quantitative trait locus cqSCN-006 alters the expression of a γ-SNAP protein. Mol. Plant. Microbe. Interact. 34, 1433–45. doi: 10.1094/MPMI-07-21-0163-R
Campbell, B. W., Hofstad, A. N., Sreekanta, S., Fu, F., Kono, T. J. Y., O’Rourke, J. A., et al. (2016). Fast neutron-induced structural rearrangements at a soybean NAP1 locus result in gnarled trichomes. Theor. Appl. Genet. 129, 1725–38. doi: 10.1007/s00122-016-2735-x
Chen, L., Cai, Y., Liu, X., Guo, C., Sun, S., Wu, C., et al. (2018). Soybean hairy roots produced in vitro by Agrobacterium rhizogenes-mediated transformation. Crop J. 6, 162–71. doi: 10.1016/j.cj.2017.08.006
Cook, D. E., Lee, T. G., Guo, X., Melito, S., Wang, K., Bayless, A. M., et al. (2012). Copy number variation of multiple genes at Rhg1 mediates nematode resistance in soybean. Science 338, 1206–9. doi: 10.1126/science.1228746
Cook, D., Bayless, A., Wang, K., Guo, X., Song, Q., Jiang, J., et al. (2014). Distinct copy number, coding sequence, and locus methylation patterns underlie Rhg1-mediated soybean resistance to soybean cyst nematode. Plant Physiol. 165, 630–47. doi: 10.1104/pp.114.235952
Davis, E. L., and Tylka, G. L. (2000). Soybean cyst nematode disease. Plant Heal. Instr. 2000, 1. doi: 10.1094/PHI-I-2000-0725-01
Deokar, A., Sagi, M., Daba, K., and Tar’an, B. (2019). QTL sequencing strategy to map genomic regions associated with resistance to ascochyta blight in chickpea. Plant Biotechnol. J. 17, 275–88. doi: 10.1111/pbi.12964
Diers, B. W., and Arelli, P. R. (1999). Management of parasitic nematodes of soybean through genetic resistance. Proc. World Soybean Res. Conf. VI 1999, 300–5. doi: 10.1038/nature05805
Dobbels, A. A., Michno, J. M., Campbell, B. W., Virdi, K. S., Stec, A. O., Muehlbauer, G. J., et al. (2017). An induced chromosomal translocation in soybean disrupts a KASI ortholog and is associated with a high-sucrose and low-oil seed phenotype. G3 7, 1215–23. doi: 10.1534/g3.116.038596
El Kasmi, F., Krause, C., Hiller, U., Stierhof, Y. D., Mayer, U., Conner, L., et al. (2013). SNARE complexes of different composition jointly mediate membrane fusion in Arabidopsis cytokinesis. Mol. Biol. Cell 24, 1593–601. doi: 10.1091/mbc.E13-02-0074
FAOSTAT (2020). Crops. Rome: FAO. Available online at: http://www.fao.org/faostat/en/#data/QC
Fekih, R., Takagi, H., Tamiru, M., Abe, A., Natsume, S., Yaegashi, H., et al. (2013). MutMap+: genetic mapping and mutant identification without crossing in rice. PLoS One 8:e68529. doi: 10.1371/journal.pone.0068529
Giovannoni, J. J., Wing, R. A., Ganal, M. W., and Tanksley, S. D. (1991). Isolation of molecular markers from specific chromosomal intervals using DNA pools from existing mapping populations. Nucleic Acids Res. 19, 6553–8. doi: 10.1093/nar/19.23.6553
Guan, R., Qu, Y., Guo, Y., Yu, L., Liu, Y., Jiang, J., et al. (2014). Salinity tolerance in soybean is modulated by natural variation in GmSALT3. Plant J. 80, 937–50. doi: 10.1111/tpj.12695
Hartman, G. L., West, E. D., and Herman, T. K. (2011). Crops that feed the World 2. Soybean-worldwide production, use, and constraints caused by pathogens and pests. Food Secur. 3, 5–17. doi: 10.1007/s12571-010-0108-x
Hill, J. T., Demarest, B. L., Bisgrove, B. W., Gorsi, B., Su, Y.-C., and Yost, H. J. (2013). MMAPPR: mutation mapping analysis pipeline for pooled RNA-seq. Genome Res. 23, 687–97. doi: 10.1101/gr.146936.112
Jeong, N., Suh, S. J., Kim, M. H., Lee, S., Moon, J. K., Kim, H. S., et al. (2012). Ln is a key regulator of leaflet shape and number of seeds per pod in soybean. Plant Cell 24, 4807–18. doi: 10.1105/tpc.112.104968
Koenning, S. R., and Wrather, J. A. (2010). Suppression of soybean yield potential in the continental United States by plant diseases from 2006 to 2009. Plant Heal. Prog. 11:5. doi: 10.1094/PHP-2010-1122-01-RS
Lakhssassi, N., Liu, S., Bekal, S., Zhou, Z., Colantonio, V., Lambert, K., et al. (2017). Characterization of the Soluble NSF Attachment Protein gene family identifies two members involved in additive resistance to a plant pathogen. Sci. Rep. 7:45226. doi: 10.1038/srep45226
Langmead, B., and Salzberg, S. L. (2012). Fast gapped-read alignment with Bowtie 2. Nat. Methods 9, 357–9. doi: 10.1038/Nmeth.1923
Li, Y. H., Reif, J. C., Jackson, S. A., Ma, Y. S., Chang, R. Z., and Qiu, L. J. (2014). Detecting SNPs underlying domestication-related traits in soybean. BMC Plant Biol. 14:251. doi: 10.1186/s12870-014-0251-1
Li, Y., Shi, X., Li, H., Reif, J. C., Wang, J., Liu, Z., et al. (2016). Dissecting the genetic basis of resistance to soybean cyst nematode combining linkage and association mapping. Plant Genome 9, 1–11. doi: 10.3835/plantgenome2015.04.0020
Li, Y., Wang, Z., Jiao, G., and Chang, R. (1991). Studies on resistance of soybean germplasm resources to race 4 of soybean cyst nematode. Sci. Agric. Sin. 24, 64–9. doi: 10.1186/s12864-015-1800-1
Lian, Y., Guo, J., Li, H., Wu, Y., Wei, H., Wang, J., et al. (2017). A new race (X12) of soybean cyst nematode in China. J. Nematol. 49, 321–6. doi: 10.21307/jofnem-2017-079
Lindner, H., Raissig, M. T., Sailer, C., Shimosato-Asano, H., Bruggmann, R., and Grossniklaus, U. (2012). SNP-Ratio Mapping (SRM): identifying lethal alleles and mutations in complex genetic backgrounds by next-generation sequencing. Genetics 191, 1381–6. doi: 10.1534/genetics.112.141341
Liu, S., Ge, F., Huang, W., Lightfoot, D. A., and Peng, D. (2019). Effective identification of soybean candidate genes involved in resistance to soybean cyst nematode via direct whole genome re-sequencing of two segregating mutants. Theor. Appl. Genet. 132, 2677–87. doi: 10.1007/s00122-019-03381-6
Liu, S., Kandoth, P. K., Lakhssassi, N., Kang, J., Colantonio, V., Heinz, R., et al. (2017). The soybean GmSNAP18 gene underlies two types of resistance to soybean cyst nematode. Nat. Commun. 8:14822. doi: 10.1038/ncomms14822
Liu, S., Kandoth, P. K., Warren, S. D., Yeckel, G., Heinz, R., Alden, J., et al. (2012). A soybean cyst nematode resistance gene points to a new mechanism of plant resistance to pathogens. Nature 492, 256–60. doi: 10.1038/nature11651
Luo, H., Pandey, M. K., Khan, A. W., Wu, B., Guo, J., Ren, X., et al. (2019). Next-generation sequencing identified genomic region and diagnostic markers for resistance to bacterial wilt on chromosome B02 in peanut (Arachis hypogaea L.). Plant Biotechnol. J. 17, 2356–69. doi: 10.1111/pbi.13153
McKenna, A., Hanna, M., Banks, E., Sivachenko, A., Cibulskis, K., Kernytsky, A., et al. (2010). The genome analysis toolkit: a map reduce framework for analyzing next-generation DNA sequencing data. Genome Res. 20, 1297–303. doi: 10.1101/gr.107524.110
Michelmore, R. W., Paran, I., and Kesseli, R. V. (1991). Identification of markers linked to disease-resistance genes by bulked segregant analysis: a rapid method to detect markers in specific genomic regions by using segregating populations. Proc. Natl. Acad. Sci. U S A. 88, 9828–32. doi: 10.1073/pnas.88.21.9828
Niblack, T. L., Lambert, K. N., and Tylka, G. L. (2006). A model plant pathogen from the kingdom animalia: Heterodera glycines, the soybean cyst nematode. Annu. Rev. Phytopathol. 44, 283–303. doi: 10.1146/annurev.phyto.43.040204.140218
Pandey, M. K., Khan, A. W., Singh, V. K., Vishwakarma, M. K., Shasidhar, Y., Kumar, V., et al. (2017). QTL-seq approach identified genomic regions and diagnostic markers for rust and late leaf spot resistance in groundnut (Arachis hypogaea L.). Plant Biotechnol. J. 15, 927–41. doi: 10.1111/pbi.12686
Patil, G. B., Lakhssassi, N., Wan, J., Song, L., Kahil, S. S., Piya, S., et al. (2019). Whole genome re-sequencing reveals the impact of the interaction of copy number variants of the rhg1 and Rhg4 genes on broad-based resistance to soybean cyst nematode. Plant Biotechnol. J. 17, 1595–1611. doi: 10.1111/pbi.13086
Ping, J., Liu, Y., Sun, L., Zhao, M., Li, Y., She, M., et al. (2014). Dt2 is a gain-of-function MADS-domain factor gene that specifies semideterminacy in soybean. Plant Cell 26, 2831–42. doi: 10.1105/tpc.114.126938
Schapire, A. L., Voigt, B., Jasik, J., Rosado, A., Lopez-Cobollo, R., Menzel, D., et al. (2008). Arabidopsis synaptotagmin 1 is required for the maintenance of plasma membrane integrity and cell viability. Plant Cell 20, 3374–88. doi: 10.1105/tpc.108.063859
Schmitt, D., and Shannon, J. (1992). Differentiating soybean responses to Heterodera glycines races. Crop Sci. 32, 275–7. doi: 10.2135/cropsci1992.0011183X003200010056x
Shaibu, A. S., Li, B., Zhang, S., and Sun, J. (2020). Soybean cyst nematode-resistance: gene identification and breeding strategies. Crop J. 8, 892–904. doi: 10.1016/j.cj.2020.03.001
Shannon, J., Arelli, P., and Young, L. (2004). “Breeding for soybean cyst nematode resistance,” in Biology and Management of Soybean Cyst Nematode, eds D. Schmitt, J. Wrather, and R. Riggs (Mareline: Schmidt & Associates of Marceline), 155–80. !
Shi, Z., Liu, S., Noel, J., Arelli, P., Meksem, K., and Li, Z. (2015). SNP identification and marker assay development for high-throughput selection of soybean cyst nematode resistance. BMC Genomics 16:314. doi: 10.1186/s12864-015-1531-3
Singh, V. K., Khan, A. W., Saxena, R. K., Kumar, V., Kale, S. M., Sinha, P., et al. (2016). Next-generation sequencing for identification of candidate genes for Fusarium wilt and sterility mosaic disease in pigeonpea (Cajanus cajan). Plant Biotechnol. J. 14, 1183–94. doi: 10.1111/pbi.12470
Song, J., Li, Z., Liu, Z., Guo, Y., and Qiu, L. J. (2017). Next-generation sequencing from bulked-segregant analysis accelerates the simultaneous identification of two qualitative genes in soybean. Front. Plant Sci. 8:919. doi: 10.3389/fpls.2017.00919
Südhof, T. C. (2013). Neurotransmitter release: the last millisecond in the life of a synaptic vesicle. Neuron 80, 675–90. doi: 10.1016/j.neuron.2013.10.022
Sun, L., Miao, Z., Cai, C., Zhang, D., Zhao, M., Wu, Y., et al. (2015). GmHs1-1, encoding a calcineurin-like protein, controls hard-seededness in soybean. Nat. Genet. 47, 939–43. doi: 10.1038/ng.3339
Tian, Y., Liu, B., Shi, X., Reif, J., Guan, R., Li, Y., et al. (2019). Deep genotyping of the gene GmSNAP facilitates pyramiding resistance to cyst nematode in soybean. Crop J. 7, 677–84. doi: 10.1016/j.cj.2019.04.003
Tran, D. T., Steketee, C. J., Boehm, J. D., Noe, J., and Li, Z. (2019). Genome-wide association analysis pinpoints additional major genomic regions conferring resistance to soybean cyst nematode (Heterodera glycines Ichinohe). Front. Plant Sci. 10:401. doi: 10.3389/fpls.2019.00401
Vickers, C. E., Schenk, P. M., Li, D., Mullineaux, P. M., and Gresshoff, P. M. (2007). pGFPGUSPlus, a new binary vector for gene expression studies and optimising transformation systems in plants. Biotechnol. Lett. 29, 1793–6.
Vuong, T. D., Sleper, D. A., Shannon, J. G., and Nguyen, H. T. (2010). Novel quantitative trait loci for broad-based resistance to soybean cyst nematode (Heterodera glycines Ichinohe) in soybean PI 567516C. Theor. Appl. Genet. 121, 1253–66. doi: 10.1007/s00122-010-1385-7
Keywords: soybean cyst nematode resistance, bulked segregant analysis, candidate genes, diagnostic markers, GmSNAP, soybean (Glycine max L. Merrill)
Citation: Shaibu AS, Zhang S, Ma J, Feng Y, Huai Y, Qi J, Li J, Abdelghany AM, Azam M, Htway HTP, Sun J and Li B (2022) The GmSNAP11 Contributes to Resistance to Soybean Cyst Nematode Race 4 in Glycine max. Front. Plant Sci. 13:939763. doi: 10.3389/fpls.2022.939763
Received: 09 May 2022; Accepted: 15 June 2022;
Published: 04 July 2022.
Edited by:
Magdalena Arasimowicz-Jelonek, Adam Mickiewicz University, PolandReviewed by:
Lingan Kong, Institute of Plant Protection (CAAS), ChinaAboozar Soorni, Isfahan University of Technology, Iran
Copyright © 2022 Shaibu, Zhang, Ma, Feng, Huai, Qi, Li, Abdelghany, Azam, Htway, Sun and Li. This is an open-access article distributed under the terms of the Creative Commons Attribution License (CC BY). The use, distribution or reproduction in other forums is permitted, provided the original author(s) and the copyright owner(s) are credited and that the original publication in this journal is cited, in accordance with accepted academic practice. No use, distribution or reproduction is permitted which does not comply with these terms.
*Correspondence: Junming Sun, sunjunming@caas.cn; Bin Li, libin02@caas.cn
†These authors have contributed equally to this work