- 1Key Laboratory of Northeast crop physiology ecology and cultivation, Ministry of Agriculture and Rural Affairs of The People’s Republic of China, Jilin Academy of Agriculture Science, Changchun, China
- 2Department of Plant and Environmental Sciences, Faculty of Science, University of Copenhagen, Taastrup, Denmark
Global rising atmospheric CO2 concentration ([CO2]) and drought stress exert profound influences on crop growth and yield. The objective of the present study was to investigate the responses of leaf gas exchange and plant water use efficiency (WUE) of wheat (C3) and maize (C4) plants to progressive drought stress under ambient (a[CO2], 400 ppm) and elevated (e[CO2], 800 ppm) atmospheric CO2 concentrations. The fraction of transpirable soil water (FTSW) was used to evaluate soil water status in the pots. Under non-drought stress, e[CO2] increased the net photosynthetic rate (An) solely in wheat, and dry matter accumulation (DMA), whereas it decreased stomatal conductance (gs) and water consumption (WC), resulting in enhanced WUE by 27.82% for maize and 49.86% for wheat. After onset of progressive soil drying, maize plants in e[CO2] showed lower FTSW thresholds than wheat, at which e.g. gs (0.31 vs 0.40) and leaf relative water content (0.21 vs 0.43) starts to decrease, indicating e[CO2] conferred a greater drought resistance in maize. Under the combination of e[CO2] and drought stress, enhanced WUE was solely found in wheat, which is mainly associated with increased DMA and unaffected WC. These varied responses of leaf gas exchange and WUE between the two species to combined drought and e[CO2] suggest that specific water management strategies should be developed to optimize crop WUE for different species in a future drier and CO2-enriched environment.
Introduction
With global climate change, agricultural systems will be facing a more changeable environment, such as the rising atmospheric CO2 levels ([CO2]) and more intense and frequent drought events (Lara and Andreo, 2011). According to IPCC projections, the annual rising rate of [CO2] has been accelerated from approximately 0.6 ppm (parts per million)/yr in the 1960 s to about 2.3 ppm/yr (2009-2018), and atmospheric [CO2] will continue to rise from currently 411.29 ppm to 800 ppm by 2100 (Kadam et al., 2014). Thus, field crops probably face the situation with elevated [CO2] (e[CO2]) together with drought stress occurring simultaneously in future climate. Thus, a better knowledge about how plants respond to drought stress under e[CO2] is required for sustainable crop productivity.
Accumulated evidence has shown e[CO2] exerts profound impacts on plant growth, phenotypic plasticity, and physiological metabolism (Leakey et al., 2009; Abebe et al., 2016). It has been well documented that plants grow under e[CO2] directly stimulate photosynthetic CO2 fixation by the increase of Rubisco carboxylation rates and decreasing rates of photorespiration (Kaminski et al., 2014), thus benefiting plant biomass accumulation, productivity, and grain yield (Leakey et al., 2009; Li et al., 2019a), despite acclimation of photosynthetic capacity is observed. However, owing to the variation of CO2-fixation efficiency between C3 and C4 species, it seems that the enhancement of carbon assimilation is more beneficial in C3 plants than these C4 plants in e[CO2] illustrated by a meta-analysis in a total of 124 plant species (Zhang et al., 2018). However, differential responses to e[CO2] between genotypes (Lecain et al., 2003), CO2 levels (Hao et al., 2016), and their interactions (Fang et al., 2019) have also been noticed. On the other hand, the negative effect of e[CO2] on plant growth has also been observed in recent studies, e.g. altering the rhizosphere environment by changing the quantity and composition of root exudates and limiting the N bioavailability for plant uptake (Feng et al., 2015); reducing the mass flow of nutrients through the soil to plant due to decreased stomatal conductance and transpiration (Wang et al., 2018). Obviously, these effects caused by e[CO2] would modulate the carbon and nitrogen metabolism in plant, which may consequently affect WUE. Yet, the underlying mechanisms influencing WUE between C3 and C4 species under e[CO2] remain largely elusive and merit further studies.
Nowadays, the impact of drought stress on crop growth and yield becomes more significant due to global environmental change (Liu et al., 2005; Vialet-Chabrand and Lawson, 2020). It has been well documented that drought stress could induce a range of morphological, physiological transcriptome and cellular changes in plants (Farooq et al., 2009), e.g. cause leaf stomatal closure, reduce plant root and leaf water relations, decrease plant gas exchange rate, or change stomatal behavior, thus directly or indirectly affect plant WUE (Aditi Gupta and Caño-Delgado, 2020). While for a long-term, drought induced adaptation responses include reducing stomatal densities, inhibiting plant growth and development, or even leading to plant death (Arnao and Hernandez-Ruiz, 2014; Shen et al., 2016). Therefore, the negative impacts of drought stress generally depend on the severity of stress and the plant growth stage, as well as plant species and genotypes (Li et al., 2022).
Recent evidence shows that plants respond to multiple stresses differently from how they do to individual stresses (Fan et al., 2020). Earlier studies have demonstrated that multiple drought and e [CO2] could reduce the stomatal conductance by modulating stomatal morphology and behavior, thereby decreasing leaf gas-exchange rates while enhancing instantaneous WUE in short term (Tubiello and Ewert, 2002; Haworth et al., 2016). It is well known that the drought-induced stomatal closure is closely linked to the levels of leaf signaling substance-abscisic acid (ABA) (Davies et al., 2002; Liu and Stutzel, 2002). While in a long term, e[CO2] and drought stress could decrease stomatal density, and alter the water balance of plants, sap flow and intrinsic water use efficiency, where both ABA signal and leaf turgor are involved and play critical role in regulation those responses (Wei et al., 2022).
At the leaf scale, WUE is primarily related to CO2 fixed in photosynthesis (An) and the amounts of water loss from the leaf interior to the atmosphere (Bertolino et al., 2019). More evidences showed both drought stress and e[CO2] had profound effects on plant stomata morphology (Zhu et al., 2018a), such as guard cell shape, stomatal density, and stomatal size, all of which relate to stomata behavior, and consequently influence plant An, gs and plant WUE (Birami et al., 2020; Jalakas et al., 2021). Leaf stomata, which primarily regulated by guard cells plays a key role in regulating water use and carbon assimilation (Buckley, 2019). In drought stressed environment, guard cells on plant leaf can directly sense the reduced hydraulic conductivity and increased [ABA] in xylem cavitation (He et al., 2019), thus decrease guard cell turgor pressure, result in the decease of stomatal pore aperture and gs, which in turn decrease the rates of CO2 uptake for carbon assimilation and water loss, and ultimately influence WUE (Ainsworth and Rogers, 2007; Hao et al., 2016). e[CO2] can cause increase in An, result in partial stomata closure in short term and reductions of stomatal density in long term, thereby decreasing gs and evapotranspiration, thus benefiting for improving plant WUE (Damour et al., 2010; Knipfer et al., 2020). However, the varied physiological and morphological response to [CO2] between specific plant species were observed. More evidences showed e[CO2] exhibited a great enhancement of An and obvious reduction for gsin C3 plants, thus contributed to an obvious increase in WUE (Ullah et al., 2019; Avila et al., 2020). While in C4 plants, e[CO2] has little or even no effects on An, and lower deduction in gs, thus results in a distinct response of WUE from C3 plants in theory (Yang et al., 2022). While plant WUE in canopy level is also associated with the plant leaf area, which directly influence plant transpiration rate (Gao and Tian, 2019). Evidences demonstrated e[CO2] plants usually have a larger leaf area, therefore leading to an increase of water loss (Conley et al., 2001), which in turn may offset the improved plant WUE in e[CO2](Henry et al., 2019). Recent studies have observed C3 plants grown in e[CO2] had a larger leaf area index increment than those C4 plants (Kirschbaum and Mcmillan, 2018; Qi et al., 2019). Therefore, whether the plant WUE in the combination of drought and e[CO2] condition is enhanced is still in doubt.
To investigate how plant gas exchange and WUE of whole plant level responded to e[CO2] and long-term drought stress, a progressive soil drying experiment with two plant species of wheat (C3) and maize was conducted under two CO2 levels of 400 ppm and 800 ppm in the climatic controlled greenhouse. We hypothesized that the change of An, gs and plant canopy leaf area, as well as biomass, are not synchronously responded to e[CO2], which may contribute to a variation in water consumption and WUE.
Materials and method
Experimental design and growth conditions
Seeds of wheat (cv. Gladius) and a wild type of ABA-deficient maize (Vp5) (provided by the Maize Genetics and Genomics Database, USA (MaizeGDB) were used in this study.
This study was conducted at the University of Copenhagen (Taastrup Campus), Denmark, on July 2, 2020. The potted plants were all grown in a temperature-controlled greenhouse. Seeds were placed in a 4 liter pot (diameter 15.2 cm and 25 cm deep) filled with 2.4 kg peat (Plugg-och Såjord Dry matter approximately 80 kg m-3, organic matter > 95%, EC 2.5-3.5 ms cm-1, pH 5.5-6.5). In total, 96 pots were set up. Eight grains of wheat and two grains of corn after surface disinfected were planted in each pot, and tap water was used for irrigation. After seeding, half of the pots were placed into a glasshouse cell with ambient CO2 concentration (400 ppm, a[CO2]), and the other half were placed in another glasshouse cell with high CO2 concentration (800 ppm, e[CO2]).
In each cell, 96 pots (48 wheat and 48 corn) were randomly distributed on a growth panel, and the CO2 was enriched inside the cell via emitting pure CO2 at one point from a bottle holder and distributed through the ventilation system. The ambient [CO2] was monitored every 6 seconds using a CO2 transmitter (GMT220 series, Vaisala Group, Helsinki, Finland). Climate conditions in two greenhouse cells were placed at: 25/16 ± 2°C (day/night) air temperature, relative humidity of 65 ± 2.5%, photoperiod of 16 h, and photosynthetic active radiation (PAR) at > 250 μmol m-2 s-1 is provided via sunlight plus LED lamps (Figure 1). Climate data is monitored every five minutes periods and recorded by the climate computer. Daily mean [CO2], relative humidity, and air temperature in the greenhouse cells throughout the experimental period are presented in Figure 1. Two weeks after sowing, corn and wheat plants were thinned in two for wheat and one for maize in each pot, and applied 1.0 g N, 0.7 g P, and 0.8 g K each pot to avoid nutrient deficiency.
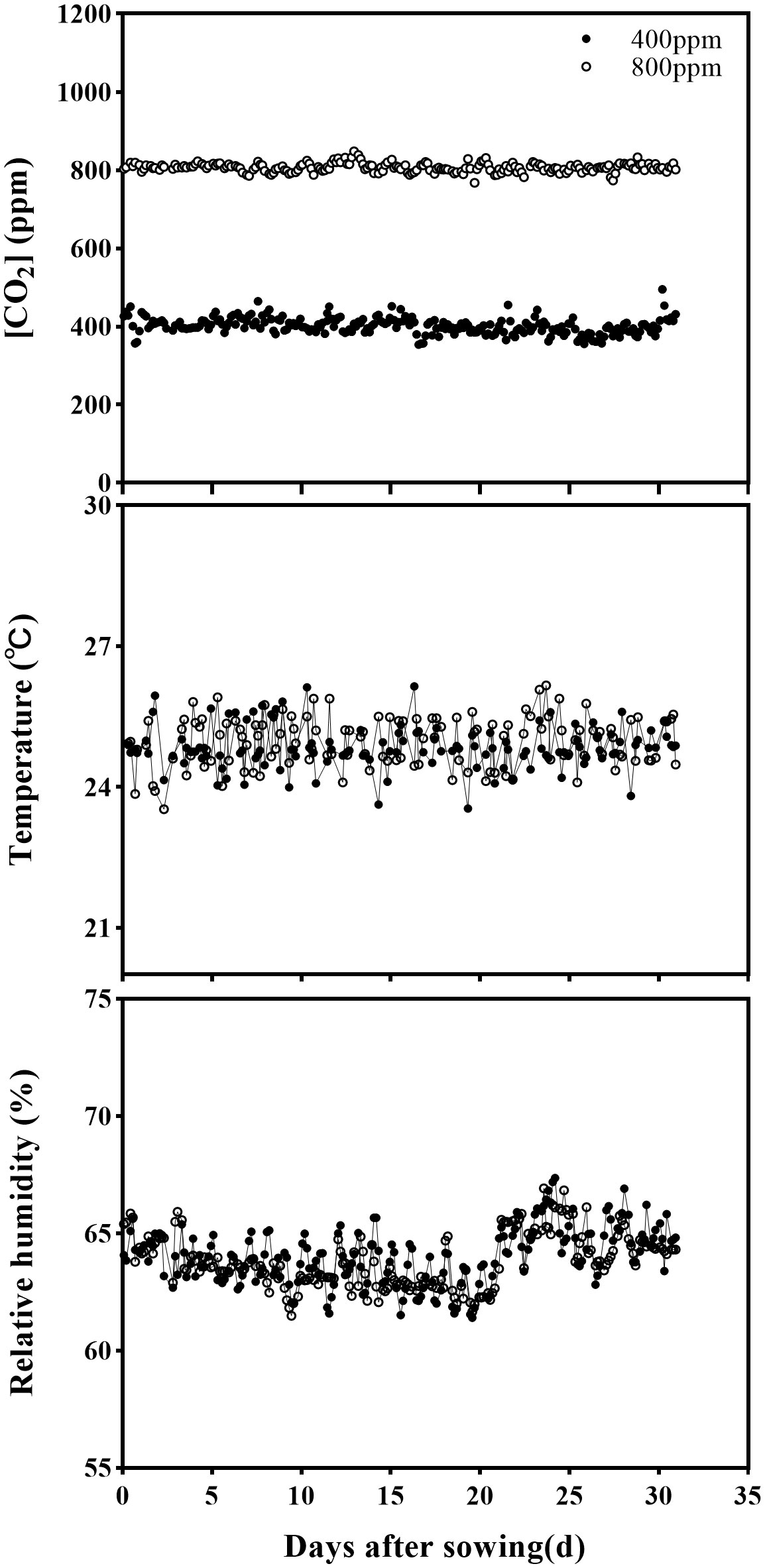
Figure 1 The average of [CO2] concentration, temperature (T), and relative humidity (RH) in greenhouse cells designated at 400 and 800 ppm during the experimental period.
After 2 weeks, half of the wheat and maize seedlings in each cell were subjected to progressive soil drying, and the remaining pots were irrigated daily to maintain 95% water holding capacity (WHC) as the control. The water content in the pot was expressed as the fraction of water in the soil that can transpire (FTSW). Total transpirable soil water (TTSW) is the difference between pot weight at 95% WHC (approximate pot weight 3.5 kg) and when the transpiration rate of the stressed plants is reduces to 10% WHC for with control plants. The daily value of FTSW is estimated as the ratio of the amount of transpiration remaining soil water in the pot to the TTSW:
where WTn is the actual pot weight at a given day and WTf is the pot weight at the time when the transpiration rate of stressed plants is 10% compared with control plants (pot weight is about 3.1 kg). The actual pot weight of the pots is obtained by weighing the pots daily during the drying cycle, and the daily transpiration is also determined. The changes in the FTSW during the experiment are shown in Figure 2.
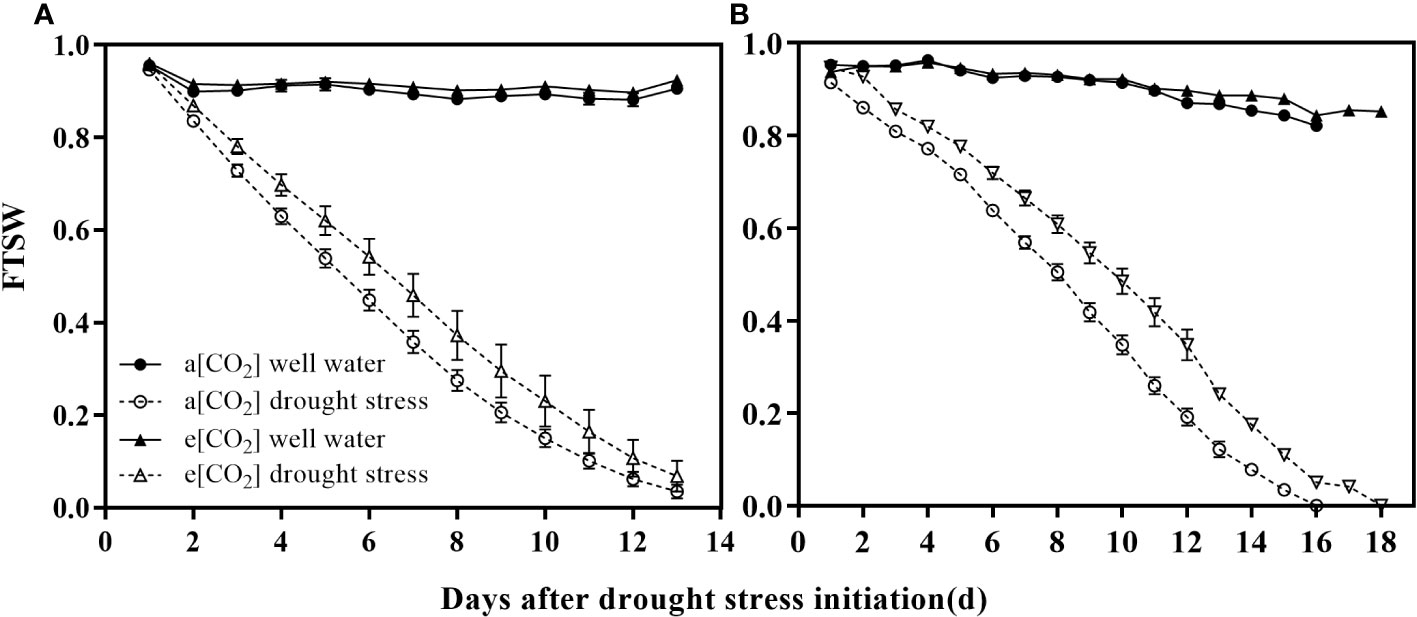
Figure 2 Trends of the fraction of transpirable soil water (FTSW) over time for well-watered and drought-stressed pots in which (A) wheat and (B) maize were grown under ambient (400 ppm, a [CO2]) and elevated (800 ppm, e [CO2]) atmospheric CO2 concentrations during progressive soil drying. Error bars indicate the standard error of the means (S.E.) (n = 4).
Sampling and measurement
After water stress, net photosynthetic rate (An) and stomatal conductance (gs) were measured daily on newly fully developed leaves above the canopy leaf from 9:00 am to 11:00 am using the LI-6400 XT handheld Photosynthesis System (LiCor Inc., Lincoln, NE, USA). During continuous soil drying, plants from each treatment were harvested five times in different soil water status; and for each species at each harvest, eight plants (4 well-watered plants, WW; and 4 drought-stressed plants, DS) were harvested, and leaf water potential (ΨLeaf) was measured with a pressure chamber (Soil Moisture Equipment Corp., SantaBarbara, CA, USA) on fully developed canopy leaves at 10:00 AM - 11:00 AM, and the rest of the leaf were immediately used for leaf relative water content determination (LRWC) and leaf ABA concentration ([ABA]leaf). The LRWC=[(FW-DW)/(SW-DW) × 100%, where FW, DW, SW indicate the fresh weight, dry weight, and saturated weight of the leaf. The [ABA]leaf was determined following the method of Liu et al., 2005. Total leaf area (LA) was measured with a LI-3100 acreage meter (Li-Cor, inc. Lincoln, Nebraska USA), and shoot dry mass (DM) was determined after 72 h of oven drying at 75°C.
Statistical analysis
Data were analyzed statistically using Microsoft Excel and SPSS 16.0 software (SPSS Inc., Chicago, IL, USA). The effects of water stress (water), ambient CO2 concentration (CO2), and their interactions (water × CO2) on the variables were analyzed using analysis of variance (ANOVA). The difierences between treatments was considered significant when the P-value was less than 0.05 in the Tukey’s HSD test.
The responses of An, gs, Ψleaf, and LRWC to soil drying were described using a linear plateau model (Faralli et al., 2019):
If FTSW > C; y=yinitial
If FTSW < C, y= yinitial
+S×(FTSW-C) where y means An, gs, Ψleaf and LRWC, and yinitial means Anmax, gmax Ψmax and LRWCmax, respectively; C is the FTSW threshold at which y begins to deviate from the yinitial for An, gs, Ψleaf and LRWC (denoted as CA, Cg, CΨ and CLRWC, respectively). Parameters y and C were estimated by PROC NLIN of PC SAS 9.4 (SAS Institute Inc., Cary, NC, USA, 2002-2012) and the coefficient of determination (r2) was calculated. Statistical comparison of each parameter obtained from the linear-plateau regression between treatments of [CO2] or plant species was performed by t-test using MedCalcstatistical 19.0.7 software.
The relationship between increment of gs during progressive soil drying and leaf [ABA] was evaluated by linear regression. R2 of the regression lines were calculated and the statistical differences on the slopes of the regression lines between gs and leaf [ABA] in wheat and corn plants under a[CO2] and e[CO2] were performed separately by analysis of covariance (ANCOVA, WU as covariate).
Results
Net photosynthetic rate (An) and stomatal conductance (gs)
The change of net photosynthetic rate (An) and stomatal conductance (gs) under a[CO2] and e[CO2] were shown in Figure 3. Under non-stressed condition, An of wheat plant grown under e[CO2] was 22.46 μmol m-2 s-1 and 24.91% higher than those grown under a[CO2] (Figure 3A), while no differences for maize (Figure 3B). During progressive soil drying, An under e[CO2] started to decline at a significant lower FTSW threshold (Cg) than that under a[CO2] (i.e., 0.33 vs 0.56) (Figure 3A; Table 1).
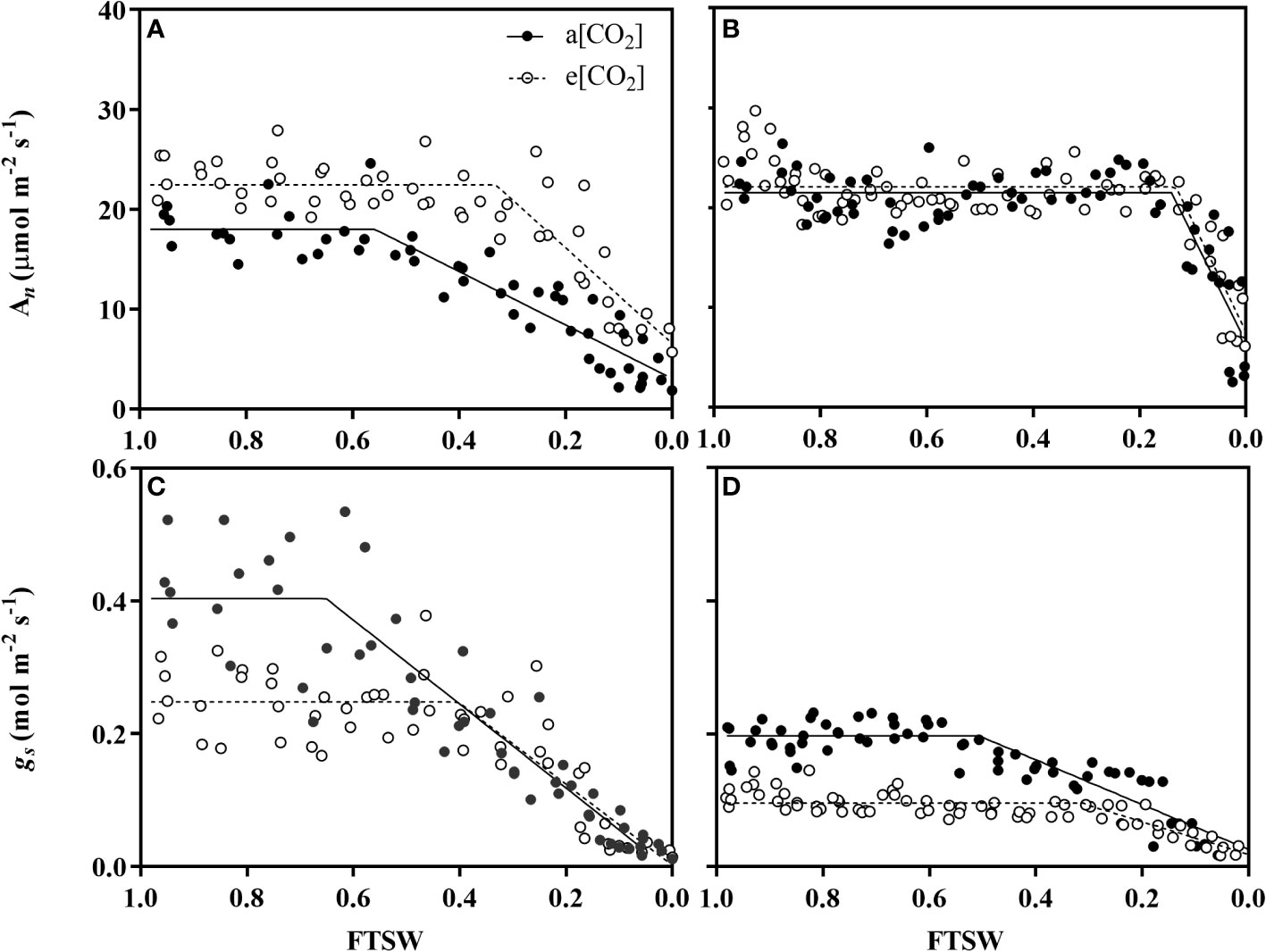
Figure 3 Changes of net photosynthesis rate (An) of (A) wheat and (B) maize plants and stomatal conductance (gs) of (C) wheat and (D) maize plants grown under ambient (400 ppm, a[CO2]) and elevated (800 ppm, e[CO2]) atmospheric CO2 concentrations during progressive soil drying. Closed and open circles indicate plants at a[CO2] and e[CO2] concentration, respectively. Error bars indicate standard error of the means (SE) (n = 4).
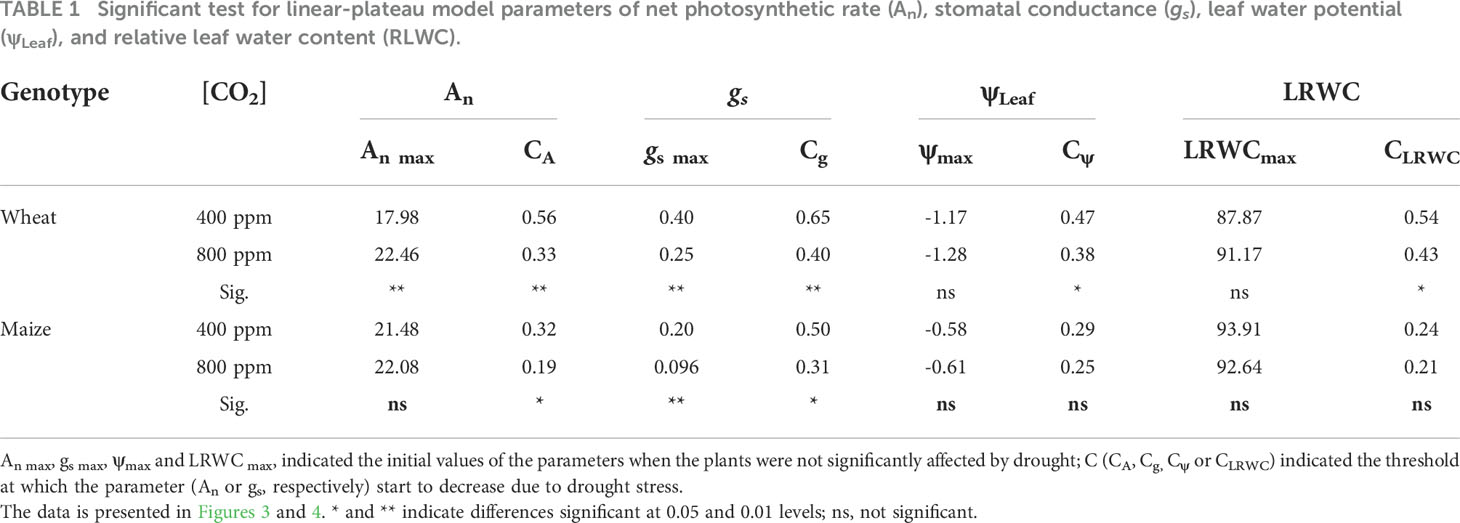
Table 1 Significant test for linear-plateau model parameters of net photosynthetic rate (An), stomatal conductance (gs), leaf water potential (ψLeaf), and relative leaf water content (RLWC).
During the initiation time of drought stress, both wheat and maize plant grown under e[CO2] had lower gs value than those grown under a[CO2](Figures 3C, D). gs under e[CO2] started to decline at a significant lower FTSW threshold (Cg) than that under a[CO2] (i.e., 0.40 vs 0.65 for wheat, and 0.31 vs 0.50 for maize) during progressive soil drying (Figures 3C, D; Table 1). The gs max of wheat plants were 0.25 μmol m-2 s-1 and 37.5% lower than that plants grown under a[CO2], and gs max of maize plants were 0.096 μmol m-2 s-1 and decreased by 52.0% (Table 1), correspondingly.
Leaf water relation
During the initiation time of drought stress, no significance differences of ψmax and LRWCmax were observed under e[CO2] and a[CO2] for either wheat nor maize plants (Figure 4; Table 1). During the progressive soil drying, e[CO2] plants showed significant lower Cψ (Figure 4A; Table 1) and CLRWC (Figure 4C; Table 1) than those a[CO2] plants for wheat, while no differences between a[CO2] and e[CO2] for maize (Figures 4B, D; Table 1).
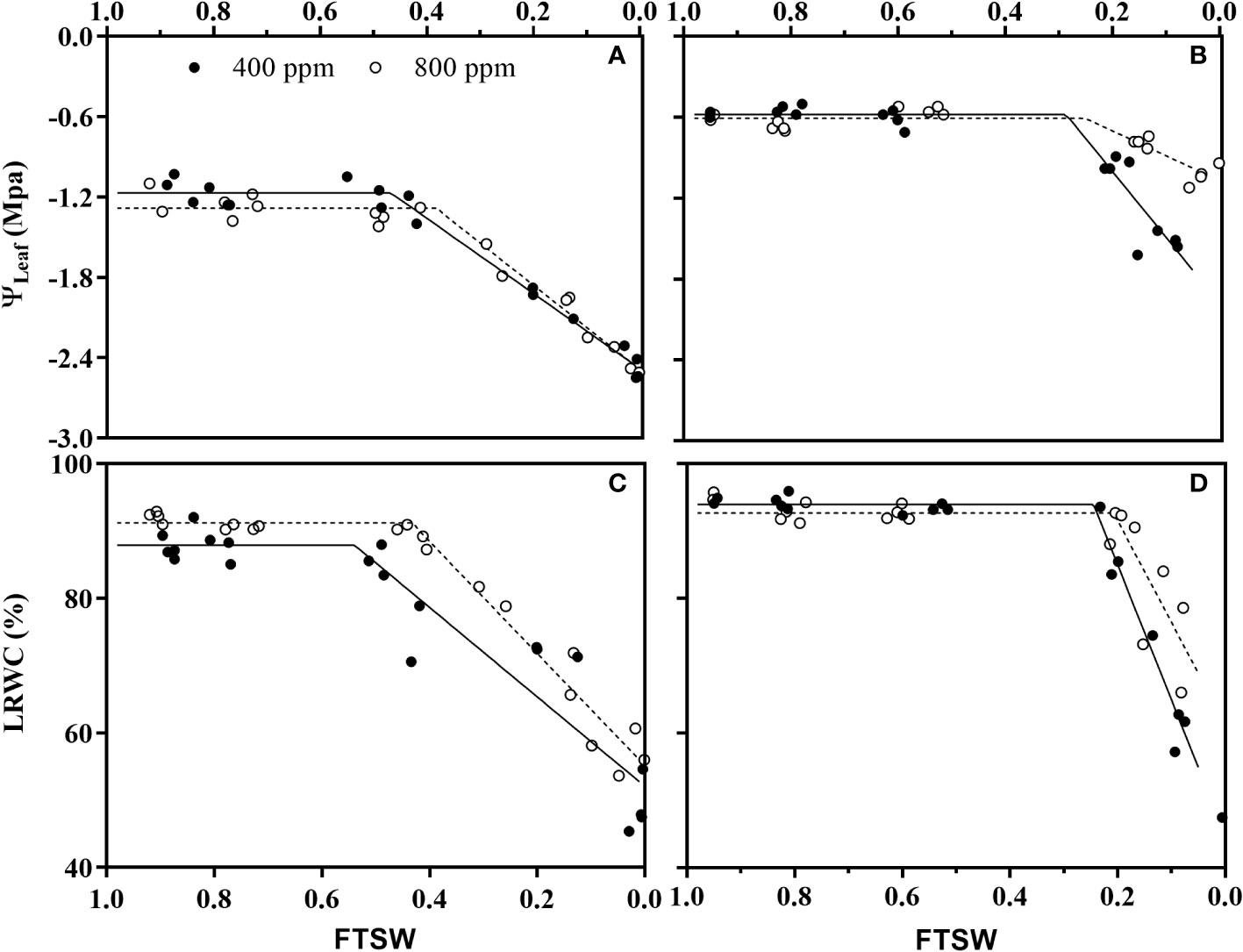
Figure 4 Changes of leaf water potential (ψLeaf) of (A) wheat and (B) maize plants, and relative leaf water content (RLWC) of (C) wheat and (D) maize plants grown under ambient (400 ppm, a[CO2]) and elevated (800 ppm, e[CO2]) atmospheric CO2 concentrations during progressive soil drying. Closed and open circles indicate plants at a[CO2] and e[CO2] concentration, respectively.
Leaf area
For wheat, LA was significantly affected by drought stress (Water), while not affected by CO2 concentration (CO2) and the interaction (Water × CO2). Under DS, the LA of e[CO2] plants 27.09% higher than a[CO2] plants (Figure 5A), while no different between e[CO2] and a[CO2] under WW condition.
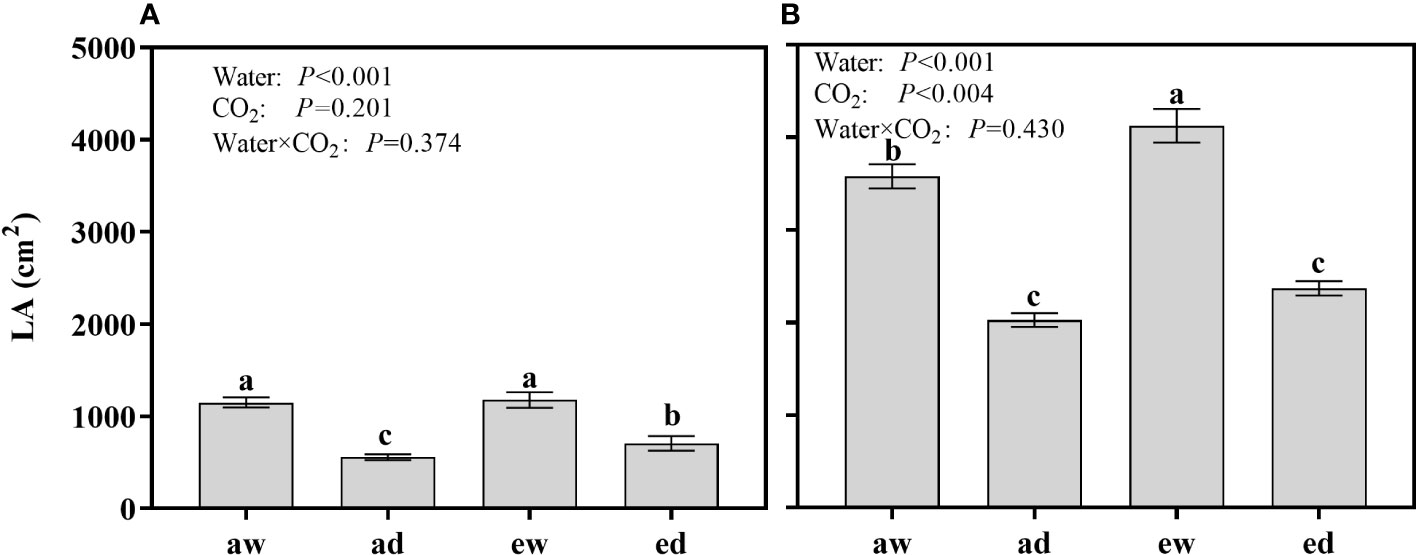
Figure 5 Changes of leaf area (LA) of (A) wheat and (B) maize plants grown under ambient (400 ppm, a[CO2]) and elevated (800 ppm, e[CO2]) atmospheric CO2 concentrations during progressive soil drying. Error bars indicate standard error of the means (SE) (n = 4). The different small letters among treatments in the figure means differences significant at the 0.01 level.
For maize, LA was significantly affected by DS and environmental CO2 (Figure 5B). DS plants had lower LA as expected, while e[CO2] showed an opposite effect. Under WW condition, LA of e[CO2] plants increased 15.22% than a[CO2] plants, while differences were not significant under DS.
Water consumption, dry matter accumulation and water use efficiency
The WC and DMA from the initiation of drought stress to final sample harvest were found significant affected by both DS and environmental CO2 (Figures 6A–D). For WC, both wheat and maize under WW in e[CO2] showed significantly higher WC compared with a[CO2] plants (Figures 6A, B), while no differences were observed between e[CO2]and a[CO2] plants under DS. For DAM, e[CO2] enhanced DMA under both WW and DS condition for wheat plants (Figure 6C), whereas the difference of DMA for maize only observed under WW condition in e[CO2] plants (Figure 6D).
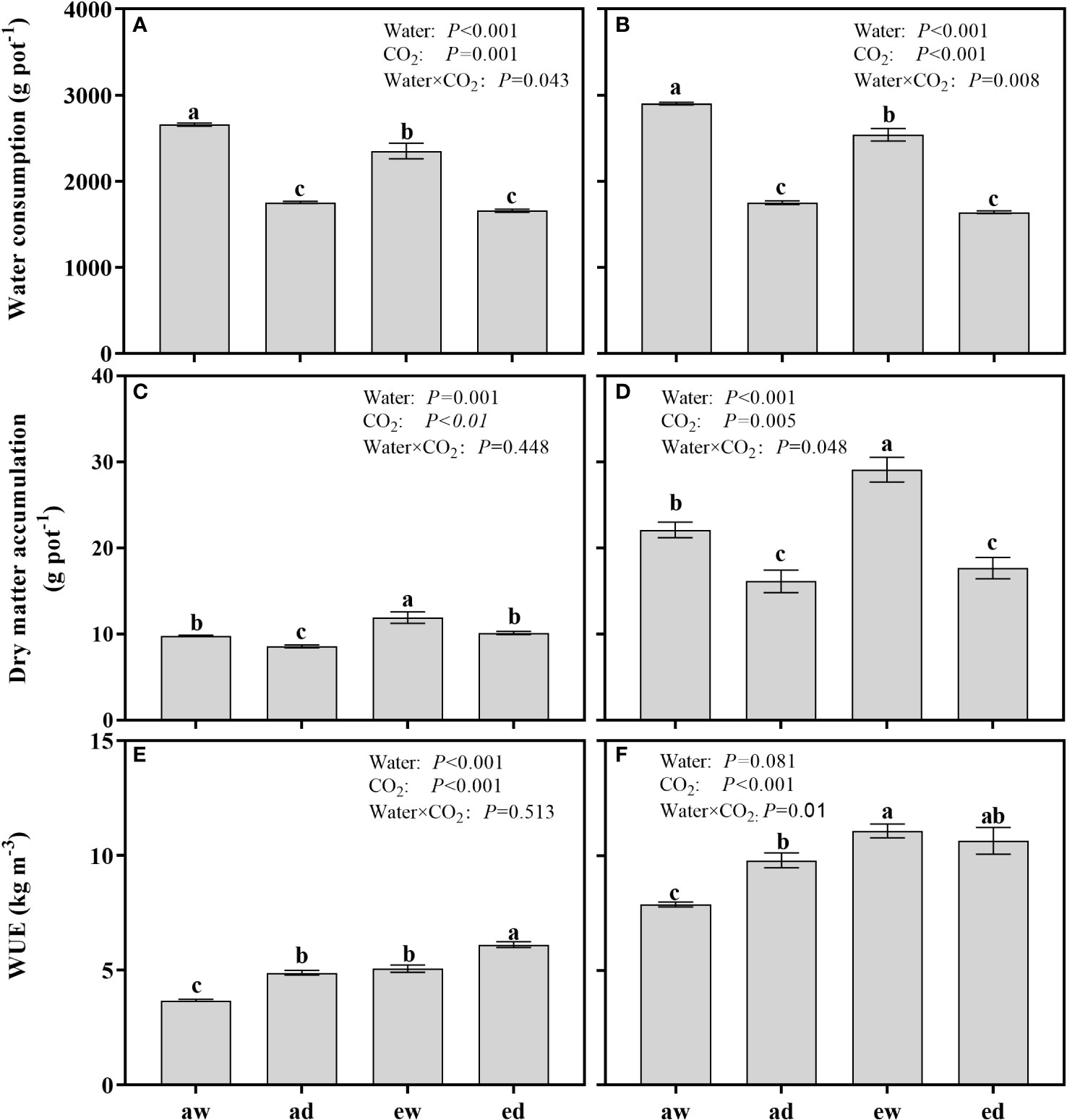
Figure 6 Comparison of water consumption (WC), dry matter accumulation(DMA), and water use efficiency (WUE) of wheat (A, C, E) and maize (B, D, F) plants grown under ambient (400 ppm, a[CO2]) and elevated (800 ppm, e[CO2]) atmospheric CO2 concentrations during progressive soil drying. Error bars indicate standard error of the means (SE) (n = 4). The different small letters among treatments in the figure means differences significant at the 0.01 level.
For WUE, both DS and e[CO2] significant enhanced WUE in wheat plant (Figure 6E). Compared to WW plants, WUE of a[CO2] and e[CO2] were enhanced by 32.83% and 22.55% under DS, respectively. In maize plants, (Figure 6F) the WUE were significantly affected by environmental CO2 and the interaction of DS and environmental CO2 (Water × CO2). Compared to a[CO2], e[CO2] plants under WW and DS condition were enhanced by 39.29% and 8.74%, respectively.
Leaf ABA concentration during progressive drought stress
DS and environmental CO2 significant affected the ABA concentration in leaf ([ABA]leaf) (Figure 7). For WW plants (aw and ew), [ABA]leaf in both wheat (Figure 8A) and maize plant (Figure 8B) had lower value, while showed an opposite trend in DS (ad and ed). [ABA]leaf in DS increased exponentially during progressive soil drying for both wheat and maize plants grown under both CO2 environments. In addition, for maize plant, e[CO2] showed higher [ABA]leaf relative to the a[CO2] plants (Figure 7B), while significant higher [ABA]leaf only be observed during the final stage for wheat plant.
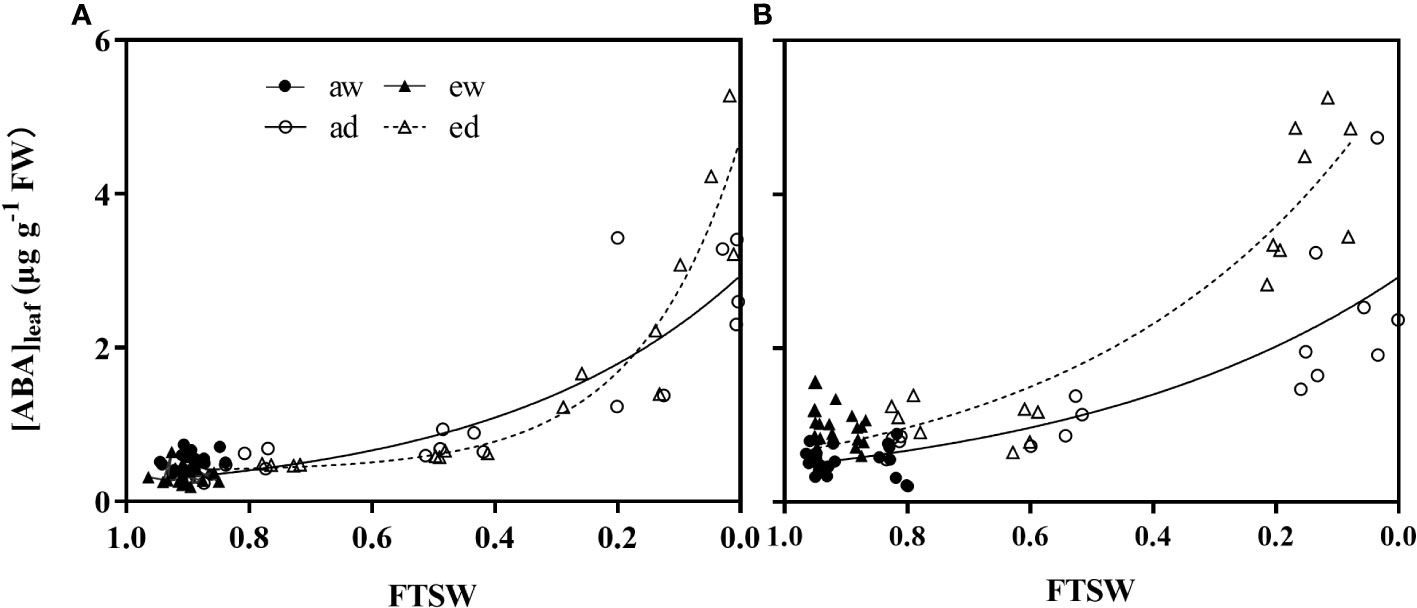
Figure 7 Changes of leaf ABA concentration of (A) wheat and (B) maize plants under ambient (400 ppm, a[CO2 ]) and elevated (800 ppm, e[CO2 ]) atmospheric CO2 concentrations during progressive soil drying.
To further understand how gs response to [ABA]leaf during the progressive soil drying, the linear regression analysis were conducted and presented in Figures 8A, B. gs decreased linearly with the increase of [ABA]leaf for both plant genotypes and environmental CO2, and the intercepts of the regression lines were significantly differed between the two CO2 environments for both plants, while significant differences of the slope of the regression lines between a[CO2] and e[CO2] was only observed for maize plants.
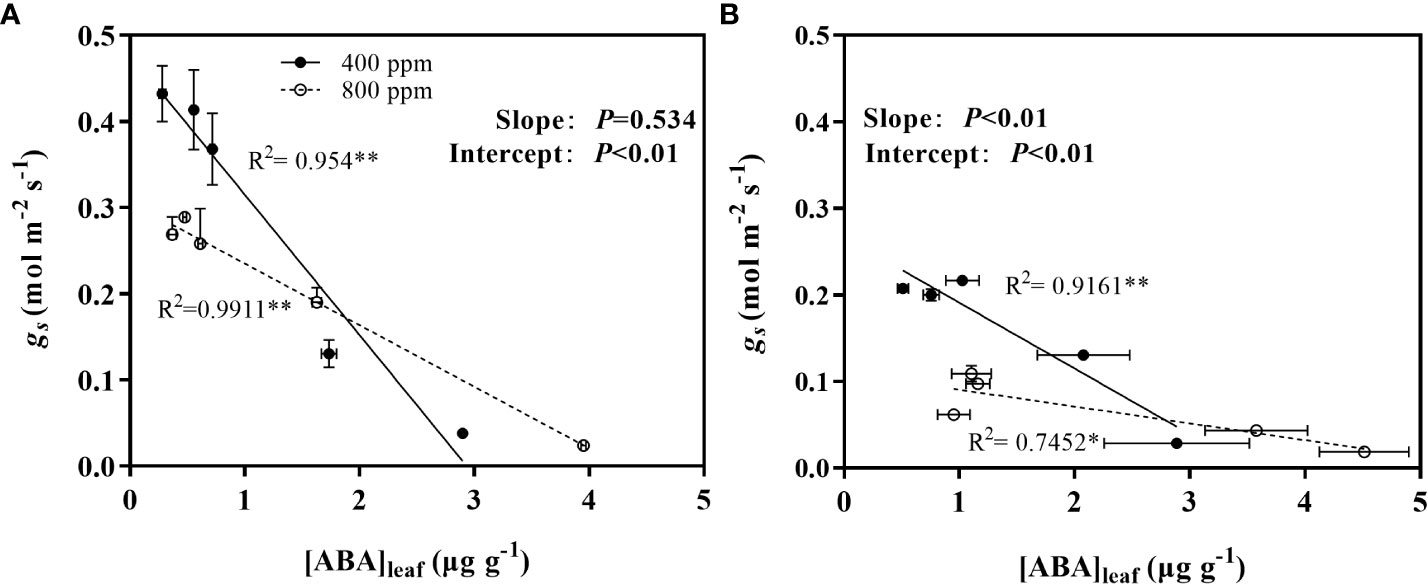
Figure 8 Relations between leaf ABA concentration ([ABA]leaf) with stomatal conductance (gs)of wheat (A) and maize (B) plants grown under ambient (400 ppm) and elevated (800 ppm) atmospheric CO2 concentrations during progressive soil drying. Error bars indicate standard error of the means (SE) (n = 4).
Discussion
Atmospheric Carbon dioxide (CO2) is an essential substrate for photosynthesis, due to the diffidence of the CO2-concentrating mechanism between C3 and C4 plants (Kadam et al., 2014; Liu et al., 2019), plants with C3 photosynthetic pathway grown in e[CO2] mostly exhibit greater enhancement of An than those with a C4 pathway (Prior et al., 2011). Consistent with this, wheat plants in e[CO2] treatment under the non-drought stress conditions, exhibited an obvious enhancement of An than those grown in a[CO2] (Figure 3A), whereas no differences were observed in maize plants (Figure 3B). This varied response of An to e[CO2] between wheat plant and maize plant could be attributed to the CO2 concentrate in the bundle sheath cell, elevated CO2 concentration can induce C3 plants to intake more CO2 molecules thus stimulating leaf photosynthesis (Allen et al., 2011; Liu et al., 2019). Moreover, during the progressive soil drying, the FTSW threshold of An for the wheat plant in e[CO2] is 0.33, which is significantly lower than that of in a[CO2] with 0.56. The retarded responses of An to soil drying under e[CO2] for wheat plants again confirmed e[CO2] that induced plant drought adaption, and similar results have been confirmed by the recent study of Yang et al., 2022 in tomato, as well as Wei et al., 2022 in amaranth and maize.
Stomata plays a central role in controlling plant CO2 and H2O exchange between the interior of leaf and the exterior environment (Lawson, 2009), which is very sensitive to the exterior environment, such as environmental [CO2] and water status changes (Mcainsh and Taylor, 2017; Zhu et al., 2018a). In the present study, it was observed that the gs in both plant species were lower in e[CO2], compared to a[CO2], the results are in agreement with the common conclusion that e[CO2] could decrease the gs by modulating the stomatal aperture in a short term effect. Interestingly, compared to maize, a more pronounced reduction of gsmax was found in wheat plants (Figures 3C, D), This is in agreement with the common conclusion that plants grown in e[CO2] generally caused a great reduction in gs for C3 plants than C4 species, which also indicates that C4 plant may have a higher environmental [CO2] tolerance than C3 plants in environmental climate change.
Generally, e[CO2] reduces leaf gs and consequently regulates CO2 and H2O exchange between plants and the atmosphere, thus influencing leaf water relations (Qiu et al., 2008; Birami et al., 2020). In the present study, e[CO2] had no effect on leaf water potential (ψLeaf) and LRWC during the onset of the drought stress (Figure 4). The similar results were also found in tomato (Wei et al., 2018), wheat and other plant species (Li et al., 2019b), and this could be attributed to plant’s morphological and physiological adaptation strategies to conserve leaf water balance for survival, such as decreasing transpiration rate by stomatal regulation (Chavan et al., 2019), enhancing photosynthetic enzyme activities for more non-structural carbohydrate concentration (e.g. sucrose, hexose, starch), and higher water use efficiency (Liu et al., 2004; Li et al., 2019b). Additionally, under drought stress condition, the threshold of LRWC and ψLeaf for both species in e[CO2] were significantly lower than those plants grown in a[CO2], which indicates the plants grown in e [CO2] retarded the response of plants to soil water deficit. And this e[CO2]-induced response is possibly associated with “hydroactive feedback”, which involved abscisic acid (ABA) production in plant leaves (Liu et al., 2003; Li et al., 2020).
Stomatal regulation also plays an important role in influencing plant growth and water consumption (Mcainsh and Taylor, 2017). Early studies illustrated that e[CO2] could decrease the gs by modulating the stomatal movement (e.g. stomatal aperture (SA) in a short term or changing stomatal morphology (e.g. stomatal size and stomatal density (SD) in a long time (Xu et al., 2016; Mcainsh and Taylor, 2017). Hence, the combination of e[CO2] and drought-induced stomata response could reduce the gs and subsequent leaf water status, thereby decreasing water consumption by modulating plant morphology, physiology, and biochemistry response (Qiu et al., 2008; Birami et al., 2020). As expected, under non-drought stress condition, both species at e[CO2] showed lower water consumption than those plants grown in a[CO2], the positive linear relationship between water consumption and gs (figure not showed) indicating that the water consumption is primarily related to the gs changes.
While on the whole-plant level, the plant water consumption is not only related to gs changes, but is also associated with the stomatal morphology (Peters et al., 2018), and factors that influence the crop canopy evapotranspiration (Xu et al., 2016), such as leaf area, leaf temperature, vapour pressure deficit (VPD) in a long-term effect (Jalakas et al., 2021). Evidences also showed that the “trade-offs” effect between reductions in stomatal conductance and increases in leaf area are critical to plant water consumption (Berens et al., 2019). Here, the water consumption of both species between treatments ad and ed were not significant (Figures 6A, B), the result indicating the progressive water deficit may diminish the e[CO2] induced water conservation effect due to increment of LA, together with subsequent water transpiration in wheat. This phenomenon was also confirmed in soybean using the free air CO2 enrichment method (Bernacchi et al., 2007) and other studies (Hatfield and Dold, 2019). Further, studies noticed that e[CO2] could impair the effectiveness of stomatal closure (Haworth et al., 2016), thereby weakened the conservation-effect induced by e[CO2] and resulting in inevitable water consumption to severe water deficit, thus contributing to the insignificant water consumption between treatment ad and ed for both species.
In recent years, the fraction of transpirable soil water (FTSW) is widely used to evaluate the soil water status in drought stress (Liu and Stutzel, 2002; Yang et al., 2022). In the present study, the leaf gas exchange parameters (An and gs), as well as leaf water relations (ψLeaf and LRWC) to progressive soil water deficit were estimated. It was found that both wheat and maize plants are grown in e[CO2] showed lower An, gs, ψLeaf and LRWC FTSW thresholds (except An and LRWC for maize) values (Figures 3 and 4, Table 1), suggesting that e[CO2] modulates the plant drought stress adoption. Meanwhile, FTSW thresholds of maize in DS under e[CO2] were also significantly lower than those grown in maize (eg. gs was 0.33 for wheat vs 0.19 for maize). The results indicate that the maize plants may become less sensitive to soil drying when exposed to e[CO2]. The varied response of plant genotypes or species to plant gas exchange parameters on An and gs were also consistent with the earlier studies by (M, 2015; Abebe et al., 2016) in maize and by Liu et al., 2019 in the tomato plants. The latest research also confirms our findings that the different response to drought stress and e[CO2] were also existed between C4 species amaranth (dicot) and maize (monocot) (Wei et al., 2022), although the mechanisms are still unclear.
For C3 plants, e[CO2] stimulate An leading to a CO2-fertilizing effect, thereby increasing plant growth and subsequently increased plant biomass (Lecain et al., 2003), while little or even no effects on C4 plants, such as maize and sorghum (Wang et al., 2012). Here, it was found that both wheat and maize grown in e[CO2] showed obvious increase in LA and DMA under non-drought stress (Figures 5, 6B, C), as well as maize plants under drought stress (Figures 5B and 6C), while the effect was not significant for maize in drought stress. This might be due to various adaptation strategies. Earlier studies showed that e[CO2] can regulate more carbon allocation to root, thus altering root or shoot architecture, e.g. LA, root-shoot ratio (Wullschleger et al., 2002; Zhu et al., 2018b). For maize plants, e[CO2] might regulate more production of fine roots to gather scarce water for progressive soil drying. However, more evidence in root traits should be investigated.
Plant WUE is an early response indicator for evaluating the physiological or ecological response to environmental change (Hatfield and Dold, 2019). In most cases, e[CO2] could improve the leaf-level WUE due to the decreased leaf transpiration rate by moderating gs, while no effect on leaf photosynthesis under mild and moderate drought stress (Oliveira et al., 2016; Zhang et al., 2018), while for a long-term effect, evidence showed that that drought stress has a stronger impact on gs than e [CO2] (Ullah et al., 2019; Li et al., 2020), thus result in a complex response. In present studies, under progressive drying, wheat plants grown in e[CO2] accelerated An than those in a[CO2], hereby increase DMA (Figure 6C), and further contribute to a greater WUE. While for maize plants, e[CO2] combined drought stress showed no effect on DMA (Figure 6D), due to neither DMA nor water consumption was affected by e[CO2] to progressive water status. Recent evidence shows that plants respond to multiple stresses differently from how they do to individual stresses (Berens et al., 2019), our results provided direct evidence that C3 plants and C4 plants might have different modulation mechanisms to e[CO2] and progressive water deficit. Thus, specific water management strategies should be developed to optimize crop WUE in a future global atmospheric drier and CO2-enriched.
To cope with the diverse stress conditions, plants evolved and developed various adaptation strategies response, these responses to different stresses are highly complex and may be involved in the changes at the transcriptome, cellular, and physiological levels (Vanaja et al., 2015). Stomatal closure is regarded be an essential strategy to defend against the combination of e[CO2] and drought stress. Increasing evidence showed that soil drying-induced stomatal closure is mainly regulated by the root-to-shoot ABA signal (Liu et al., 2005), and leaf turgor during severe drought (Jalakas et al., 2021). Consistent with this, the leaf ABA concentration of both species was found significantly enhanced during the final stage of drought stress (Figure 7), which confirm that the leaf ABA could act as a signal substance to participate in plants’ drought adaptation in e[CO2]. Additionally, the effect of e[CO2] on plant growth and performance is rather complex, except for its directly effect on plant carbon metabolism, but also related to air temperature by trapping heat effect (Dusenge et al., 2019). Thus, more attention about the interaction effect of e[CO2] and air temperature on plant species response should be paid in the future.
Conclusion
During the progressive soil drying, e[CO2] of both wheat and maize species exhibited lower FTSW threshold of An, gs, ψLeaf and LRWC than grown in a[CO2], indicating that e[CO2] modulate a greater plant drought stress adaptation; meanwhile, the gradually increased leaf [ABA], together with its negative linear relationship with gs were observed in both species, suggesting leaf ABA may play important role in the combination of e[CO2] and progressive drying modulated drought adaptation. To progressive soil drying, e[CO2] showed higher DMA, but no effect on LA and WC, thus attributed to higher WUE in wheat, while not affected maize WUE. Conclusively, the varied responses of leaf gas exchange and WUE to e[CO2] and soil water deficits in wheat and maize species might be due to different modulation mechanisms. Therefore, specific water management strategies should be developed to optimize crop WUE in a future global atmospheric drier and CO2-enriched.
Data availability statement
The original contributions presented in the study are included in the article/Supplementary Material. Further inquiries can be directed to the corresponding author.
Author contributions
FL designed the experiments. QC conducted the experiments and analyzed the data. QC wrote the manuscript with the help of GL and FL. All authors contributed to the article and approved the submitted version.
Funding
This work was financially supported by the Agricultural science and technology innovation project of Jilin Province CXGC201903GH.
Acknowledgments
This is a short text to acknowledge the contributions of specific colleagues, institutions, or agencies that aided the efforts of the authors.
Conflict of interest
The authors declare that the research was conducted in the absence of any commercial or financial relationships that could be construed as a potential conflict of interest.
Publisher’s note
All claims expressed in this article are solely those of the authors and do not necessarily represent those of their affiliated organizations, or those of the publisher, the editors and the reviewers. Any product that may be evaluated in this article, or claim that may be made by its manufacturer, is not guaranteed or endorsed by the publisher.
Supplementary material
The Supplementary Material for this article can be found online at: https://www.frontiersin.org/articles/10.3389/fpls.2022.953712/full#supplementary-material
Abbreviations
e[CO2], elevated atmospheric CO2 concentrations; a[CO2], ambient atmospheric CO2 concentrations; An, net photosynthetic rate; gs, stomatal conductance; ΨLeaf, leaf water potential; LRWC, leaf relative water content; DMA, dry matter accumulation; WHC, water holding capacity; WUE, water use efficiency.
References
Abebe, A., Pathak, H., Singh, S. D., Bhatia, A., Harit, R. C., Kumar, V. (2016). Growth, yield and quality of maize with elevated atmospheric carbon dioxide and temperature in north–west India. Agric. Ecosyst. Environ. 218, 66–72. doi: 10.1016/j.agee.2015.11.014
Aditi Gupta, A. R.-M., Caño-Delgado, A. I. (2020). The physiology of plant responses to drought. Science 368, 266–269. doi: 10.1126/science.aaz7614
Ainsworth, E. A., Rogers, A. (2007). The response of photosynthesis and stomatal conductance to rising [CO2]: mechanisms and environmental interactions. Plant Cell Environ. 30, 258–270. doi: 10.1111/j.1365-3040.2007.01641.x
Allen, L. H., Jr., Kakani, V. G., Vu, J. C., Boote, K. J. (2011). Elevated CO2 increases water use efficiency by sustaining photosynthesis of water-limited maize and sorghum. J. Plant Physiol. 168, 1909–1918. doi: 10.1016/j.jplph.2011.05.005
Arnao, M. B., Hernandez-Ruiz, J. (2014). Melatonin: plant growth regulator and/or biostimulator during stress? Trends Plant Sci. 19, 789–797. doi: 10.1016/j.tplants.2014.07.006
Avila, R. T., Cardoso, A. A., De Almeida, W. L., Costa, L. C., Machado, K. L. G., Barbosa, M. L., et al. (2020). Coffee plants respond to drought and elevated [CO2] through changes in stomatal function, plant hydraulic conductance, and aquaporin expression. Environ. Exp. Bot. 177, 104148. doi: 10.1016/j.envexpbot.2020.104148
Berens, M. L., Wolinska, K. W., Spaepen, S., Ziegler, J., Nobori, T., Nair, A., et al. (2019). Balancing trade-offs between biotic and abiotic stress responses through leaf age-dependent variation in stress hormone cross-talk. Proc. Natl. Acad. Sci. U.S.A. 116, 2364–2373. doi: 10.1073/pnas.1817233116
Bernacchi, C. J., Kimball, B. A., Quarles, D. R., Long, S. P., Ort, D. R. (2007). Decreases in stomatal conductance of soybean under open-air elevation of [CO2] are closely coupled with decreases in ecosystem evapotranspiration. Plant Physiol. 143, 134–144. doi: 10.1104/pp.106.089557
Bertolino, L. T., Caine, R. S., Gray, J. E. (2019). Impact of stomatal density and morphology on water-use efficiency in a changing world. Front. Plant Sci. 10, 225. doi: 10.3389/fpls.2019.00225
Birami, B., Nagele, T., Gattmann, M., Preisler, Y., Gast, A., Arneth, A., et al. (2020). Hot drought reduces the effects of elevated CO2 on tree water-use efficiency and carbon metabolism. New Phytol. 226, 1607–1621. doi: 10.1111/nph.16471
Buckley, T. N. (2019). How do stomata respond to water status? New Phytol. 224, 21–36. doi: 10.1111/nph.15899
Chavan, S. G., Duursma, R. A., Tausz, M., Ghannoum, O. (2019). Elevated CO2 alleviates the negative impact of heat stress on wheat physiology but not on grain yield. J. Exp. Bot. 70, 6447–6459. doi: 10.1093/jxb/erz386
Conley, M. M., Kimball, B., Brooks, T., Pinter, P., Jr., Hunsaker, D., Wall, G., et al. (2001). CO2 enrichment increases water-use efficiency in sorghum. New Phytol. 151, 407–412. doi: 10.1046/j.1469-8137.2001.00184.x
Damour, G., Simonneau, T., Cochard, H., Urban, L. (2010). An overview of models of stomatal conductance at the leaf level. Plant Cell Environ. 33, 1419–1438. doi: 10.1111/j.1365-3040.2010.02181.x
Davies, W. J., Wilkinson, S., Loveys, B. (2002). Stomatal control by chemical signalling and the exploitation of this mechanism to increase water use efficiency in agriculture. New Phytolt. 153, 449–460. doi: 10.1046/j.0028-646X.2001.00345.x
Dusenge, M. E., Duarte, A. G., Way, D. A. (2019). Plant carbon metabolism and climate change: elevated CO2 and temperature impacts on photosynthesis, photorespiration and respiration. New Phytol. 221 (1), 32–49. doi: 10.1111/nph.15283
Fan, X., Cao, X., Zhou, H., Hao, L., Dong, W., He, C., et al. (2020). Carbon dioxide fertilization effect on plant growth under soil water stress associates with changes in stomatal traits, leaf photosynthesis, and foliar nitrogen of bell pepper (Capsicum annuum L.). Environ. Exp. Bot. 179, 104203. doi: 10.1016/j.envexpbot.2020.104203
Fang, L., Abdelhakim, L. O. A., Hegelund, J. N., Li, S., Liu, J., Peng, X., et al. (2019). ABA-mediated regulation of leaf and root hydraulic conductance in tomato grown at elevated CO2 is associated with altered gene expression of aquaporins. Hortic. Res. 6, 104. doi: 10.1038/s41438-019-0187-6
Faralli, M., Williams, K. S., Han, J., Corke, F. M. K., Doonan, J. H., Kettlewell, P. S. (2019). Water-saving traits can protect wheat grain number under progressive soil drying at the meiotic stage: A phenotyping approach. J. Plant Growth Regul. 38, 1562–1573. doi: 10.1007/s00344-019-09956-3
Farooq, M., Wahid, A., Kobayashi, N., Fujita, D., Basra, S. M. A. (2009). “Plant drought stress: Effects, mechanisms and management,” in Sustainable agriculture (Dordrecht: Springer), 153–188. doi: 10.1007/978-90-481-2666-8_12
Feng, Z., Rutting, T., Pleijel, H., Wallin, G., Reich, P. B., Kammann, C. I., et al. (2015). Constraints to nitrogen acquisition of terrestrial plants under elevated CO2. Glob. Chang. Biol. 21, 3152–3168. doi: 10.1111/gcb.12938
Gao, J., Tian, K. (2019). Stem and leaf traits as co-determinants of canopy water flux. Plant Divers. 41, 258–265. doi: 10.1016/j.pld.2019.06.003
Hao, X. Y., Li, P., Li, H. Y., Zong, Y. Z., Zhang, B., Zhao, J. Z., et al. (2016). Elevated CO2 increased photosynthesis and yield without decreasing stomatal conductance in broomcorn millet. Photosynthetica 55, 176–183. doi: 10.1007/s11099-016-0226-6
Hatfield, J. L., Dold, C. (2019). Water-use efficiency: Advances and challenges in a changing climate. Front. Plant Sci. 10, 103. doi: 10.3389/fpls.2019.00103
Haworth, M., Killi, D., Materassi, A., Raschi, A., Centritto, M. (2016). Impaired stomatal control is associated with reduced photosynthetic physiology in crop species grown at elevated [CO2]. Front. Plant Sci. 7, 1568. doi: 10.3389/fpls.2016.01568
Henry, C., John, G. P., Pan, R., Bartlett, M. K., Fletcher, L. R., Scoffoni, C., et al. (2019). A stomatal safety-efficiency trade-off constrains responses to leaf dehydration. Nat. Commun. 10 (1), 1–9. doi: 10.1038/s41467-019-11006-1
He, W., Yoo, G., Moonis, M., Kim, Y., Chen, X. (2019). Impact assessment of high soil CO2 on plant growth and soil environment: a greenhouse study. Peer J. 7, e6311. doi: 10.7717/peerj.6311
Jalakas, P., Takahashi, Y., Waadt, R., Schroeder, J. I., Merilo, E. (2021). Molecular mechanisms of stomatal closure in response to rising vapour pressure deficit. New Phytol. 232, 468–475. doi: 10.1111/nph.17592
Kadam, N. N., Xiao, G., Melgar, R. J., Bahuguna, R. N., Quinones, C., Tamilselvan, A., et al. (2014). Agronomic and physiological responses to high temperature, drought, and elevated CO2 interactions in cereals. Adv. Agron., 111–156. doi: 10.1016/B978-0-12-800131-8.00003-0
Kaminski, K. P., Kørup, K., Nielsen, K. L., Liu, F., Topbjerg, H. B., Kirk, H. G., et al. (2014). Gas-exchange, water use efficiency and yield responses of elite potato (Solanum tuberosum L.) cultivars to changes in atmospheric carbon dioxide concentration, temperature and relative humidity. Agric. For Meteorol. 187, 36–45. doi: 10.1016/j.agrformet.2013.12.001
Kirschbaum, M. U., Mcmillan, A.J.C.F.R. (2018). Warming and elevated CO2 have opposing influences on transpiration. which is more important? Curr. For Rep. 4, 51–71. doi: 10.1007/s40725-018-0073-8
Knipfer, T., Bambach, N., Hernandez, M. I., Bartlett, M. K., Sinclair, G., Duong, F., et al. (2020). Predicting stomatal closure and turgor loss in woody plants using predawn and midday water potential. Plant Physiol. 184, 881–894.
Lara, M., Andreo, C. S. (2011). “C4 plants adaptation to high levels of CO2 and to drought environments,” in Abiotic stress in plants-mechanisms and adaptations London: IntechOpen, 415–428. doi: 10.5772/24936
Leakey, A. D., Ainsworth, E. A., Bernacchi, C. J., Rogers, A., Long, S. P., Ort, D. R. (2009). Elevated CO2 effects on plant carbon, nitrogen, and water relations: six important lessons from FACE. J. Exp. Bot. 60, 2859–2876.
Lecain, D. R., Morgan, J. A., Mosier, A. R., Nelson, J. A. (2003). Soil and plant water relations determine phot+osynthetic responses of C3 and C4 grasses in a semi-arid ecosystem under elevated CO2. Ann. Bot. 92, 41–52. doi: 10.1093/aob/mcg109
Li, P., Hao, X., Aryal, M., Thompson, M., Seneweera, S. (2019a). Elevated carbon dioxide and nitrogen supply affect photosynthesis and nitrogen partitioning of two wheat varieties. J. Plant Nutr. 42, 1290–1300. doi: 10.1080/01904167.2019.1616758
Li, X., Kristiansen, K., Rosenqvist, E., Liu, F. (2019b). Elevated CO2 modulates the effects of drought and heat stress on plant water relations and grain yield in wheat. J. Agron. Crop Sci. 205, 362–371. doi: 10.1111/jac.12330
Li, S., Li, X., Wei, Z., Liu, F. (2020). ABA-mediated modulation of elevated CO2 on stomatal response to drought. Curr. Opin. Plant Biol. 56, 174–180. doi: 10.1016/j.pbi.2019.12.002
Li, X., Palta, J. A., Liu, F. (2022). Editorial: Modulation of stomatal response by elevated CO2 in plants under drought and heat stress. Front. Plant Sci. 13, 843999. doi: 10.3389/fpls.2022.843999
Liu, F., Andersen, M. N., Jacobsen, S.-E., Jensen, C. R. (2005). Stomatal control and water use efficiency of soybean (Glycine max l. merr.) during progressive soil drying. Environ. Exp. Bot. 54, 33–40. doi: 10.1016/j.envexpbot.2004.05.002
Liu, J., Hu, T., Fang, L., Peng, X., Liu, F. (2019). CO2 elevation modulates the response of leaf gas exchange to progressive soil drying in tomato plants. Agric. Fort Meteorol. 268, 181–188. doi: 10.1016/j.agrformet.2019.01.026
Liu, F., Jensen, C. R., Andersen, M. N. (2003). Hydraulic and chemical signals in the control of leaf expansion and stomatal conductance in soybean exposed to drought stress. Funct. Plant Biol. 30, 65–73. J.F.P.B. doi: 10.1071/FP02170
Liu, F., Jensen, C. R., Andersen, M. N. (2004). Drought stress effect on carbohydrate concentration in soybean leaves and pods during early reproductive development: its implication in altering pod set. Field Crops Res. 86, 1–13. doi: 10.1016/S0378-4290(03)00165-5
Liu, F., Stutzel, H. (2002). Leaf expansion, stomatal conductance, and transpiration of vegetable amaranth (Amaranthus sp.) in response to soil drying. J. Am. Soc. Hortic. Sci. 127, 878–883. doi: 10.21273/JASHS.127.5.878
Mcainsh, M. R., Taylor, J. E. (2017). “Plant physiology and development,” in Encyclopedia of applied plant sciences, 2nd ed. London; Elsevier Ltd, 128–134.
Oliveira, V. F., Silva, E. A., Carvalho, M. (2016). Elevated CO2 atmosphere minimizes the effect of drought on the cerrado species chrysolaena obovata. Front. Plant Sci. 7. doi: 10.3389/fpls.2016.00810
Peters, W., van der Velde, I. R., Van Schaik, E., Miller, J. B., Ciais, P., Duarte, H. F., et al. (2018). Increased water-use efficiency and reduced CO2 uptake by plants during droughts at a continental-scale. Nat. Geosci. 11, 744–748. doi: 10.1038/s41561-018-0212-7
Prior, S. A., Runion, G. B., Marble, S. C., Rogers, H. H., Gilliam, C. H., Torbert, H. A. (2011). A review of elevated atmospheric CO2 effects on plant growth and water relations: implications for horticulture. HortScience 46, 158–162. doi: 10.21273/HORTSCI.46.2.158
Qi, W., Liu, J., Leung, F. (2019). A framework to quantify impacts of elevated CO2 concentration, global warming and leaf area changes on seasonal variations of water resources on a river basin scale. J. Hydol. 570, 508–522. doi: 10.1016/j.jhydrol.2019.01.015
Qiu, Z. B., Liu, X., Tian, X. J., Yue, M. (2008). Effects of CO2 laser pretreatment on drought stress resistance in wheat. J. Photochem. Photobiol. B Biol. 90, 17–25. doi: 10.1016/j.jhydrol.2019.01.015
Shen, J., Xiao, Q., Qiu, H., Chen, C., Chen, H. (2016). Integrative effect of drought and low temperature on litchi (Litchi chinensis sonn.) floral initiation revealed by dynamic genome-wide transcriptome analysis. Sci. Rep. 6, 32005. doi: 10.1038/srep32005
Tubiello, F. N., Ewert, F. (2002). Simulating the effects of elevated CO2 on crops: approaches and applications for climate change. Eur. J. Agron. 18, 57–74. doi: 10.1016/S1161-0301(02)00097-7
Ullah, H., Santiago-Arenas, R., Ferdous, Z., Attia, A., Datta, A. (2019). Improving water use efficiency, nitrogen use efficiency, and radiation use efficiency in field crops under drought stress: A review. Adv. Agron. 156, 109–157. doi: 10.1016/bs.agron.2019.02.002
Vanaja, M., Maheswari, M., Jyothi Lakshmi, N., Sathish, P., Yadav, S. K., Salini, K., et al. (2015). Variability in growth and yield response of maize genotypes at elevated CO2 concentration. Adv. Plants Agric. Res. 2, 0042. doi: 10.15406/apar.2015.02.00042
Vialet-Chabrand, S., Lawson, T. (2020). Thermography methods to assess stomatal behaviour in a dynamic environment. J. Exp. Bot. 71, 2329–2338. doi: 10.1093/jxb/erz573
Wang, D., Heckathorn, S. A., Wang, X., Philpott, S. M. (2012). A meta-analysis of plant physiological and growth responses to temperature and elevated CO2. Oecologia 169, 1–13. doi: 10.1007/s00442-011-2172-0
Wang, L., Yan, Y., Wang, X., Wang, T., Duan, Q., Zhang, W. (2018). Mass flow measurement of gas-liquid two-phase CO2 in CCS transportation pipelines using Coriolis flowmeters. Int. J. Greenh Gas Control 68, 269–275. doi: 10.1016/j.ijggc.2017.11.021
Wei, Z., Abdelhakim, L. O. A., Fang, L., Peng, X., Liu, J., Liu, F. (2022). Elevated CO2 effect on the response of stomatal control and water use efficiency in amaranth and maize plants to progressive drought stress. Agricl. Water Manag. 266, 107609. doi: 10.1016/j.agwat.2022.107609
Wei, Z., Du, T., Li, X., Fang, L., Liu, F. (2018). Interactive effects of CO2 concentration elevation and nitrogen fertilization on water and nitrogen use efficiency of tomato grown under reduced irrigation regimes. Agricl. Water Manag. 202, 174–182. doi: 10.1016/j.agwat.2018.02.027
Wullschleger, S. D., Tschaplinski, T. J., Norby, R. J. (2002). Plant water relations at elevated CO2–implications for water-limited environments. Plant Cell Environ. 25, 319–331. doi: 10.1046/j.1365-3040.2002.00796.x
Xu, Z., Jiang, Y., Jia, B., Zhou, G. (2016). Elevated-CO2 response of stomata and its dependence on environmental factors. Front. Plant Sci. 7, 657. doi: 10.3389/fpls.2016.00657
Yang, X., Zhang, P., Wei, Z., Liu, J., Hu, X., Liu, F. (2022). Effects of elevated CO2 and nitrogen supply on leaf gas exchange, plant water relations and nutrient uptake of tomato plants exposed to progressive soil drying. Sci. Hortic. 292, 110643. doi: 10.1016/j.scienta.2021.110643
Zhang, J., Jiang, H., Song, X., Jin, J., Zhang, X. (2018). The responses of plant leaf CO2/H2O exchange and water use efficiency to drought: A meta-analysis. Sustainability 10, 551. doi: 10.3390/su10020551
Zhu, X., Cao, Q., Sun, L., Yang, X., Yang, W., Zhang, H. (2018a). Stomatal conductance and morphology of arbuscular mycorrhizal wheat plants response to elevated CO2 and NaCl stress. Front. Plant Sci. 9, 1363. doi: 10.3389/fpls.2018.01363
Keywords: abscisic acid (ABA), climate change, elevated CO2, gas exchange, stomatal conductance
Citation: Cao Q, Li G and Liu F (2022) Elevated CO2 enhanced water use efficiency of wheat to progressive drought stress but not on maize. Front. Plant Sci. 13:953712. doi: 10.3389/fpls.2022.953712
Received: 01 June 2022; Accepted: 28 October 2022;
Published: 17 November 2022.
Edited by:
Hamada AbdElgawad, Beni-Suef University, EgyptReviewed by:
Manoj Parihar, ICAR-Vivekananda Parvatiya Krishi Anusandhan Sansthan, IndiaNieves Goicoechea, University of Navarra, Spain
Copyright © 2022 Cao, Li and Liu. This is an open-access article distributed under the terms of the Creative Commons Attribution License (CC BY). The use, distribution or reproduction in other forums is permitted, provided the original author(s) and the copyright owner(s) are credited and that the original publication in this journal is cited, in accordance with accepted academic practice. No use, distribution or reproduction is permitted which does not comply with these terms.
*Correspondence: Fulai Liu, fl@plen.ku.dk