- 1The National Forestry and Grassland Administration Engineering Research Center for Germplasm Innovation and Utilization of Warm-season Turfgrasses, Institute of Botany, Jiangsu Province and Chinese Academy of Sciences, Nanjing, China
- 2Jiangsu Coastal Area Institute of Agricultural Sciences, Yancheng, China
Zoysia matrella [L.] Merr. is one of the three most economically important Zoysia species due to its strong salt tolerance and wide application. However, the molecular mechanisms regulating salt tolerance in Z. matrella remain unknown. The protein disulfide isomerase ZmPDI of Z. matrella was obtained by salt stress screening with yeast cells, and its expression was significantly upregulated after salt stress. Based on the obtained ZmPDI overexpression transgenic Z. matrella plants, we carried out salt tolerance identification and found that ZmPDI can significantly enhance the salt tolerance of Z. matrella. Root samples of OX-ZmPDI transgenic and wild-type plants were collected at 0 and 24 h after salt treatments for RNA-seq and data-independent acquisition (DIA) proteome sequencing. Combined analysis of the transcriptome and proteome revealed that ZmPDI may enhance the salt tolerance of Z. matrella by regulating TUBB2, PXG4, PLDα2, PFK4, and 4CL1. This research presents the molecular regulatory mechanism of the ZmPDI gene in Z. matrella for resistance to salt stress and facilitates the use of molecular breeding to improve the salt tolerance of grasses.
Introduction
Saline-alkali land is widely distributed throughout the world and seriously affects the local ecological environment and economic development. How to efficiently use and improve saline-alkali land resources has become a global research hotspot. Turfgrass plays an important role in ecological and green space construction and is a pioneer plant for ameliorating soil salinization. Zoysia grass is an excellent warm-season turfgrass that is famous for its strong tolerance (including salt, cold, and drought tolerance) and wide application (including saline-alkali soil, athletic fields, home lawns, and parks) (Ge et al., 2006). The three most economically important Zoysia species are Zoysia japonica Steud., Zoysia matrella [L.] Merr. and Zoysia pacifica (Goudswaard) M. Hotta & Kuroki, and Z. matrella has the highest salt tolerance among them (Yamamoto et al., 2016). Z. matrella is a perennial warm-season turfgrass belonging to the family Gramineae, subfamily Chloridoideae, Zoysia willd (Tanaka et al., 2016a). Although the strong salt tolerance of Z. matrella is well known, the molecular mechanism is still unknown.
The current research on the salt tolerance of Z. matrella mainly focuses on the evaluation of salt tolerance and the physiological mechanism of salt tolerance. Salt tolerance evaluation shows that Z. matrella has strong salt tolerance (Marcum and Murdoch, 1994; Li et al., 2012). The physiological mechanism of salt tolerance showed that Z. matrella has large bicellular salt glands on the adaxial side and can secrete excessive Na+ from the salt glands of leaves to maintain a low Na+/K+ ratio to resist salt stress (Chen et al., 2009; Yamamoto et al., 2016). When exposed to salt stress, Z. matrella can also synthesize organic matter, such as betaine, glycine, proline, and soluble sugars, to adjust its osmotic potential to alleviate the osmotic stress caused by high salt concentrations (Li et al., 2012). Furthermore, an appropriate concentration of phosphorus under salt stress can promote root growth, increase Na+ secretion in leaves and inhibit Na+ transportation from roots to leaves, which is beneficial to the salt resistance of Z. matrella (Jiang et al., 2013).
Currently, few molecular mechanism studies on the salt tolerance of Z. matrella have been reported. A type I vacuolar H+-pyrophosphatase (VP) gene, ZmVP1, was isolated in Z. matrella, and overexpressing this gene in Arabidopsis thaliana can promote plant growth with more Na+ and K+ in the leaves and higher activities of V-ATPase and V-PPase under salt stress (Chen et al., 2015a). Chen et al. (2015b) constructed a high-quality full-length cDNA expression library in yeast using a Gateway-compatible vector system and screened 16 candidate salt-tolerant genes in Z. matrella (Chen et al., 2015b). Yeast validation experiments show that these 16 candidate salt-tolerant genes can improve the salt tolerance of yeast and exhibit different transcription levels under salt stress (Chen et al., 2015b). However, the molecular mechanism of some of these candidate salt-tolerant genes in Z. matrella, including a protein disulfide isomerase ZmPDI (NCBI accession number: KM265179), is very limited.
Protein disulfide isomerase (PDI) is a member of the thioredoxin subfamily of redox proteins and has thiol-disulfide oxidoreductase, disulfide isomerase and redox-dependent chaperone catalytic activities (Ali Khan and Mutus, 2014). PDI is mainly distributed throughout the lumen of the endoplasmic reticulum, cell surfaces and cytosol (Ali Khan and Mutus, 2014). It promotes the correct folding of proteins by catalyzing the formation of disulfide bonds and the rearrangement of mismatched disulfide bonds (Okumura et al., 2021). The functions of PDI in mammals have been extensively studied, while the functions in plants have less research. Existing studies suggest that, in Arabidopsis, AtPDI11 has been demonstrated to exhibit oxidoreductase activity in vitro (Fan et al., 2018). The AtPDI1 gene has an anti-stress function in Arabidopsis, and overexpressing AtPDI1 can increase the abiotic stress tolerance of seedlings with a higher germination ratio and longer root length (Zhang et al., 2018). Overexpression of the protein disulfide isomerase AtCYO1 has a negative effect on the initiation of chlorophyll degradation and proteolysis to maintain the functions of chloroplasts (Tominaga et al., 2018). However, studies on the regulatory mechanism of plant salt tolerance by the PDI gene have not been reported.
Previous studies showed that the heterologous expression of ZmPDI can significantly improve the salt tolerance of yeast, and the expression of ZmPDI is significantly upregulated after salt stress in Z. matrella (Chen et al., 2015b). Furthermore, ZmPDI-overexpressing transgenic Z. matrella plants have been successfully obtained (Wang K. et al., 2020). In order to further explore the regulation mechanism of ZmPDI in salt tolerance of Z. matrella, we evaluated the salt tolerance of ZmPDI-overexpressing transgenic Z. matrella plants, analyzed the gene ontology (GO) and Kyoto Encyclopedia of Genes and Genomes (KEGG) pathways that ZmPDI gene may affect after salt treatment and selected key differentially expressed genes (DEGs) that may be regulated by ZmPDI through combined transcriptome and proteome analysis. This study provides new insight into the regulatory mechanism of ZmPDI in Z. matrella for resistance to salt stress and facilitates the use of molecular breeding to improve the salt tolerance of grasses.
Materials and methods
Plant materials and treatments
The test materials were five OX-ZmPDI transgenic lines (Lines 4, 5, 7, 11, and 15) (Wang K. et al., 2020) and wild-type plants of Z. matrella, which are conserved by the Grass Research Center of Institute of Botany, Jiangsu Province and Chinese Academy of Sciences. The callus of Z. matrella was supported by Prof. Mingliang Chai from Zhejiang University (China).
Five OX-ZmPDI transgenic lines and wild-type plants were cultured in porous PVC tubes (height 40 cm, diameter 10 cm) filled with sand, and each material was cultured in 8 tubes. Water was poured into each tube material once a day, and Hoagland nutrient solution was poured and trimmed once every 5 days. When the growth was consistent, four tubes of each material were treated with NaCl solution, and the other four tubes were treated with water as the control. During salt treatment, 100 mM NaCl was used as the gradient to increase the concentration once every 3 days for progressive treatment with 400 mL each time. When the salt concentration reached 400 mM, the concentration no longer increased. Afterward, the material was treated with 400 mM NaCl every 5 days. The control and salt treatments were irrigated at the same time with 400 mL of water. Phenotypes of treated plants were observed after 30 days, and corresponding physicochemical indices of roots and leaves were determined.
The five OX-ZmPDI transgenic lines showed similar phenotypes and corresponding physicochemical index determination results, and transgenic Line 4 was selected for the next treatment and sampling. The stolons with ten nodes of OX-ZmPDI transgenic Line 4 and wild-type plants were selected and cut into small pieces with 2 nodes, and 40 small pieces of each material were planted. The hydroponic experiment was performed in the greenhouse. The hydroponic solution was 1/2 Hoagland nutrient solution, which was replaced once a week. When the growth of plants was consistent, 400 mM NaCl solution was added to the 1/2 Hoagland nutrient solution for salt treatment.
Physiological index measurement
The physicochemical indices of OX-ZmPDI transgenic lines and wild-type plants in porous PVC tubes were determined after salt treatment for 30 days. The chlorophyll contents of leaves were determined by the acetone extraction method. The free proline contents of roots were determined by the sulfosalicylic acid method. The soluble sugar contents of roots were determined by the anthrone colorimetric method. The SOD activity of roots was determined by a total superoxide dismutase kit (hydroxylamine method) (Nanjing Jiancheng Bioengineering Institute, Nanjing, China). The Na+ and K+ contents in roots and leaves were determined according to the method of Wang J. et al. (2020). All data were analyzed for variance by SPSS Statistics v.26.0 (SPSS Inc., Chicago, IL, United States) and plotted by GraphPad Prism v.8 (GraphPad Software, San Diego, CA, United States).
RNA-seq
The roots of OX-ZmPDI transgenic Line 4 and wild-type plants were sampled at 0 and 24 h after salt treatment, and three biological replicates were obtained for each sample at each time point. All samples were frozen in liquid nitrogen and stored at −80°C. Total RNA was extracted using a TRIzol reagent kit (Invitrogen, Carlsbad, CA, United States) according to the manufacturer’s protocol. A total of 12 cDNA libraries were constructed and sequenced by Gene Denovo Biotechnology Co., (Guangzhou, China) using the Illumina HiSeq2500 platform. The datasets are available in the NCBI repository http://www.ncbi.nlm.nih.gov/bioproject/PRJNA848971. De novo assembly of the Z. matrella transcriptome was accomplished via Hisat2 (v2.0.5) (Kim et al., 2015) using the Z. japonica genome as a reference (Tanaka et al., 2016b). The mapping reads of each sample were assembled using StringTie v1.3.1 (Pertea et al., 2015), and their expression abundance and variations were calculated by FPKM (fragment per kilobase of transcript per million mapped reads) values (Mortazavi et al., 2008). RNA differential expression analysis was performed by DESeq2 (Love et al., 2014) software and edgeR R package (3.18.1) (Robinson et al., 2010), which were used to analyze significant differences between two groups (determined as a p-value < 0.05 and absolute fold change ≥ 2). The DEGs were selected with a |log2FC| > 1 and false discovery rate (FDR) < 0.05.
Data-independent acquisition protein quantification
For data-independent acquisition (DIA) proteomics analysis, proteins from the root samples (the same as RNA-seqsea) were collected from OX-ZmPDI transgenic Line 4 and wild-type plants at 0 and 24 h after salt treatment. Total proteins were extracted using the cold acetone method. After grinding, each sample was dissolved in 2 mL of lysis buffer (8 M urea, 2% SDS, 1 × protease inhibitor cocktail), pyrolyzed by sonication on ice for 30 min and collected by centrifugation at 13,000 rpm for 30 min at 4°C. The supernatant was retained, and prechilled acetone was added to precipitate the proteins at −20°C overnight. After cleaning with acetone three times, the precipitants were dissolved in 8 M urea by sonication on ice. The protein concentration of each sample was determined by a BCA protein assay kit.
For each sample, 50 μg of protein was suspended in 50 μL of solution, and 1 μL of 1 M dithiothreitol (DTT) was added at 55°C for 1 h. Then, 5 μL of 20 mM indoacetamide was used to alkylate the proteins of each sample in the dark at 37°C for 1 h. Then, 300 μL of prechilled acetone was added to each sample to precipitate the proteins at −20°C overnight, and the proteins were washed twice with prechilled acetone. The proteins were resuspended with 50 mM ammonium bicarbonate and digested with sequence-grade modified trypsin (Promega, Madison, WI, United States) at a substrate/enzyme ratio of 50:1 (w/w) at 37°C for 16 h.
The spectral libraries were generated (Fang et al., 2021), and the spectrophotometer was set up to search the database of Z. matrella.1 After nano-HPLC–MS/MS analysis, the raw DIA data were acquired, processed and analyzed by Spectronaut X (Biognosys AG, Switzerland) with default parameters (Fang et al., 2021). After performing Student’s t-test, differentially expressed proteins (DEPs) were filtered with a q-value < 0.05 and |Fold change (FC)| > 1.5.
Bioinformatics analysis
The correlation analysis of replicas was performed by R. Correlation to evaluate repeatability between three biological replicate samples. Principal component analysis (PCA) was performed with the R package gmodels2 to reveal the relationship of all the samples. The correlation analysis of RNA-seq and proteome was performed by R (version 3.5.1), and a nine-quadrant map was drawn based on changes in the expression of the gene in the transcriptome and proteome. All DEP and DEG functions were annotated in the GO database, KEGG database and Clusters of Orthologous Groups of proteins (COG/KOG) database. The significantly enriched GO functions and KEGG pathways were examined within DEGs and DEPs with FDR ≤ 0.05 and q-value ≤ 0.05, respectively.
Quantitative RT-PCR validation
Twelve DEGs were randomly selected from the gene list in Table 1 for qRT–PCR validation. The primers were designed by Primer Premier 5.0 software and are listed in Supplementary Table 10. The ZjActin (GenBank: GU290545.1) gene was used as a housekeeping gene. Each sample was analyzed with three biological replicates, and qRT–PCR assays were carried out as described by Xie et al. (2015).
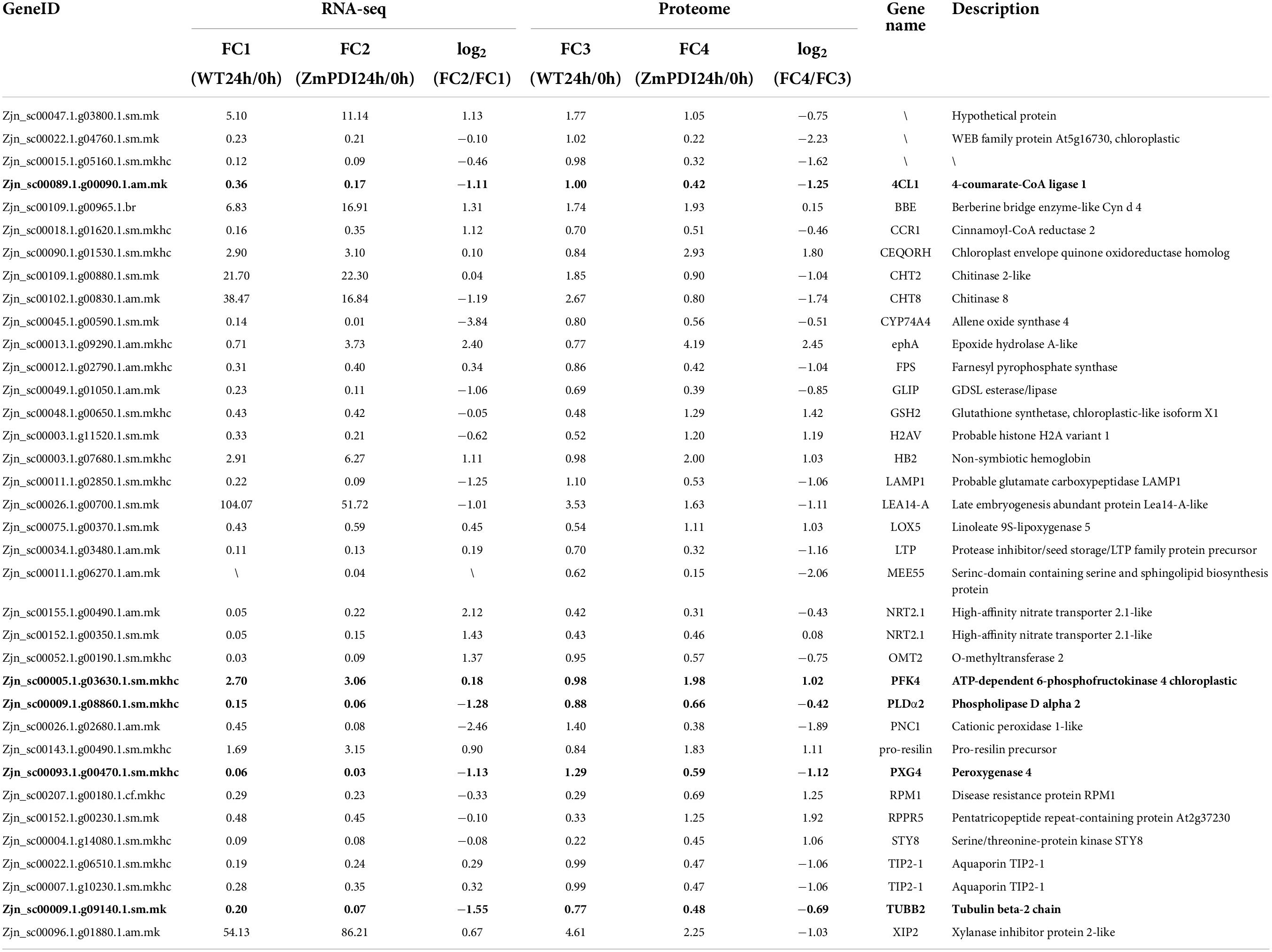
Table 1. Selected differentially expressed genes (DEGs) from transcriptome and proteome association analysis.
Results
Salt tolerance evaluation of OX-ZmPDI transgenic plants of Zoysia matrella
To evaluate the salt tolerance of OX-ZmPDI transgenic lines, 400 mM NaCl solution was applied for 30 days to treat the OX-ZmPDI transgenic lines and wild-type plants. After salt treatment, the wild-type plants were obviously withered and yellowed, while the OX-ZmPDI transgenic lines all maintained normal growth, which was not significantly different from the control (CK) treatment (Figure 1A). In the wild-type plants, the dry weights of the aboveground and underground biomasses of the salt-treated plants were significantly lower than those of the CK plants (Figures 1B,C). However, the dry weights of the aboveground and underground biomasses of the OX-ZmPDI transgenic lines were not significantly different between the salt and CK treatments (Figures 1B,C).
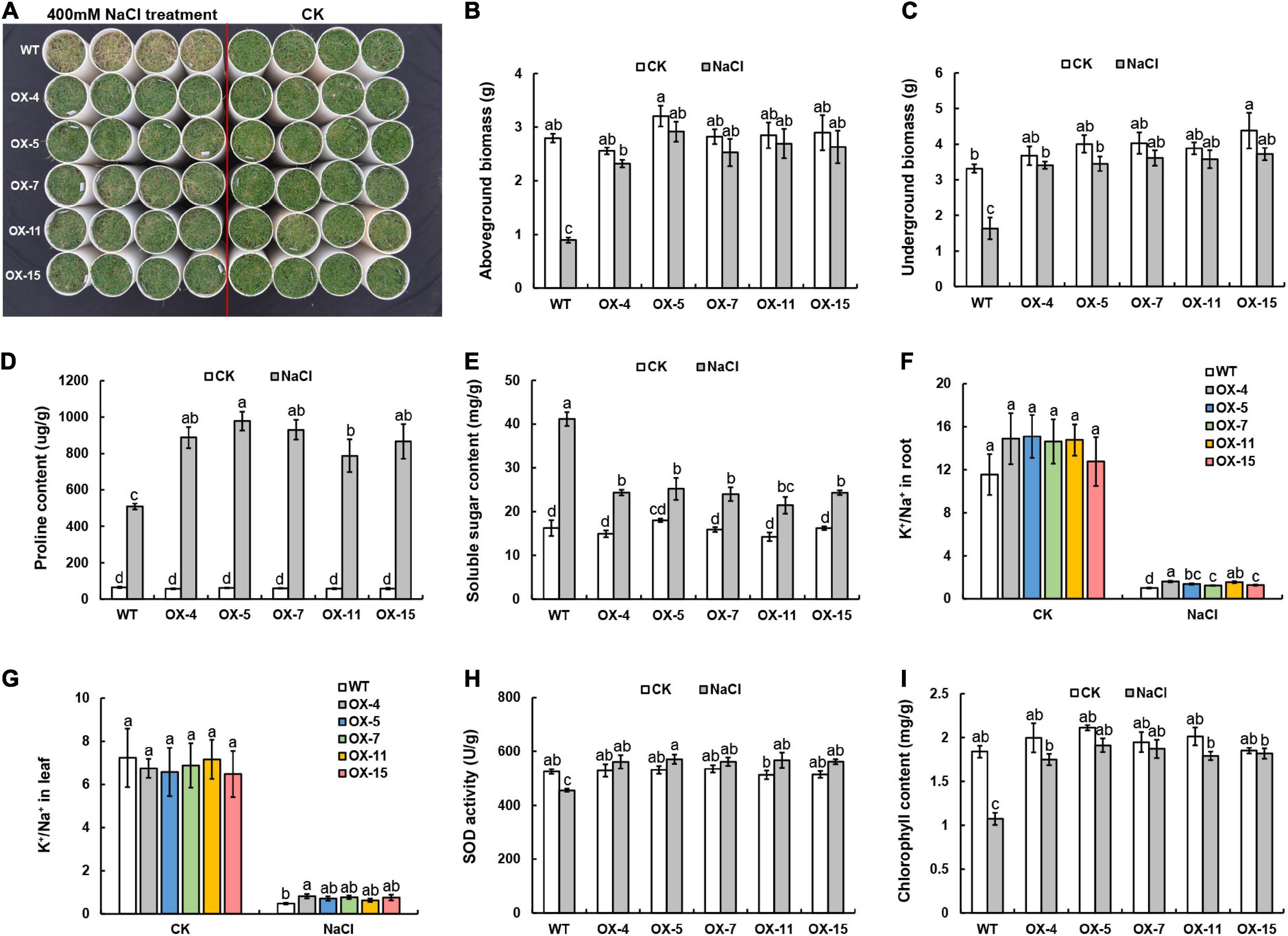
Figure 1. Phenotypic and physiological responses to salt stress in OX-ZmPDI transgenic plants of Z. matrella. (A) OX-ZmPDI transgenic lines and wild-type (WT) plants were exposed to 400 mM NaCl and control (CK) treatments for 30 days. (B) The aboveground biomasses of OX-ZmPDI transgenic and wild-type plants after NaCl and CK treatments for 30 days. (C) The underground biomasses of OX-ZmPDI transgenic and wild-type plants after NaCl and CK treatments for 30 days. (D) The proline contents of OX-ZmPDI transgenic and wild-type plants after NaCl and CK treatments for 30 days. (E) The soluble sugar contents of OX-ZmPDI transgenic and wild-type plants after NaCl and CK treatments for 30 days. (F) The K+/Na+ ratio in OX-ZmPDI transgenic and wild-type plant roots after CK and NaCl treatments. (G) The K+/Na+ ratio in OX-ZmPDI transgenic and wild-type plant leaves after CK and NaCl treatments. (H) The chlorophyll contents of OX-ZmPDI transgenic and wild-type plants after NaCl and CK treatments for 30 days. (I) The SOD activity of OX-ZmPDI transgenic and wild-type plants after NaCl and CK treatments for 30 days. Values are presented as the mean ± SE. Letters above the bars indicate significant differences between the respective values (p < 0.05).
After salt treatment, the contents of the osmotic adjustment substances, proline and sugars, were detected in OX-ZmPDI transgenic lines and wild-type plants. The proline contents of wild-type plants increased after salt treatment, and the proline contents of OX-ZmPDI transgenic lines were much higher than those of wild-type plants (Figure 1D). However, the soluble sugar contents of Z. matrella plants all increased after salt treatment, and the degree of increase in OX-ZmPDI transgenic lines was significantly lower than that in wild-type plants (Figure 1E). The K+/Na+ ratio is also an important index with which to evaluate the salt tolerance of plants. In the CK treatment, the K+/Na+ ratios in roots and leaves were not significantly different between the wild-type and OX-ZmPDI transgenic plants (Figures 1F,G). However, after treatment with 400 mM NaCl, the K+/Na+ ratios of OX-ZmPDI transgenic lines in roots were all obviously higher than those of wild-type plants, especially OX-ZmPDI transgenic line OX-4 (Figure 1F). Meanwhile, the K+/Na+ ratios in the leaves of OX-ZmPDI transgenic line OX-4 were significantly higher than those of the wild-type plants (Figure 1G). Furthermore, chlorophyll contents and superoxide dismutase (SOD) activity are also important for assessing salt tolerance. In this study, the chlorophyll content of wild-type plants was obviously lower than that of the CK treatment, while the chlorophyll contents of OX-ZmPDI transgenic lines showed no significant difference compared with the CK treatment (Figure 1H). After salt treatment, the SOD activity of wild-type plants showed an obviously downward trend, while that of OX-ZmPDI transgenic lines showed no significant difference compared with the CK treatment (Figure 1I).
Transcriptome and proteome sequencing of OX-ZmPDI transgenic plants
Previous studies show that roots may make significant contributions to the salt tolerance in zoysiagrass (Wang J. et al., 2020). In the preliminary physiological index measurement, the K+/Na+ ratios were measured both in roots and leaves. The results showed that the K+/Na+ ratios in roots of all OX-ZmPDI transgenic lines were obviously higher than those of wild-type plants, while only the K+/Na+ ratio in leaves of OX-ZmPDI transgenic line OX-4 was significantly higher than wild-type plants (Figures 1F,G). Therefore, in order to analyze the molecular mechanism by which the ZmPDI gene enhances the salt tolerance of Z. matrella, root samples of the OX-ZmPDI transgenic line OX-4 and wild-type plants were collected at 0 and 24 h after treatment with 400 mM NaCl for transcriptome and proteome sequencing. For RNA-seq, an average of 43.24 million raw reads were obtained, and 97.98% of these reads were confirmed as clean reads (Supplementary Table 1). A total of 69.05–75.64, 15.10−20.39, and 8.65–10.38% of the reads were mapped to exons, introns and intergenic regions in the reference genome, respectively (Supplementary Table 2). In total, 35,197 genes were identified by the RNA-seq assays, including 33,660 unigenes and 1,537 novel genes.
DIA technology was used to perform the comparative analysis of OX-ZmPDI transgenic line OX-4 and wild-type plants. More than half of the peptides had lengths between 7 and 18 amino acids (Supplementary Figure 1A). After filtering with FDR ≤ 0.05, 36,076 peptides and 6,842 proteins were obtained, and 5,040 (73.66%) proteins had more than one peptide (Supplementary Figures 1B,C). Among the total 6,842 proteins, 6,370 (93.10%) were annotated in at least one of the GO, KEGG and KOG protein libraries (Supplementary Figure 1D). Transcription factor analysis showed that most proteins were in the bZIP transcription factor family, followed by the C3H and Trihelix transcription factor families (Supplementary Figure 1E).
The correlation values of biological repeat samples for RNA-seq and the proteome were all above 0.866 (Supplementary Figures 2A,B). A total of 4,887, 11, 9, and 6,384 DEGs were identified in the WT24h vs. WT0h, ZmPDI0h vs. WT0h, ZmPDI24h vs. WT24h and ZmPDI24h vs. ZmPDI0h comparisons, respectively (Supplementary Figure 2C). Because the numbers of DEGs in the ZmPDI0h vs. WT0h and ZmPDI24h vs. WT24h comparisons were very low, we chose WT24h vs. WT0h and ZmPDI24h vs. ZmPDI0h comparisons to continue the further analysis, and found that the downregulation of DEGs in these two comparisons was greater than the upregulation of DEGs (Figure 2A). For proteome sequencing, 304 and 319 DEPs were identified in the WT24h vs. WT0h and ZmPDI24h vs. ZmPDI0h comparisons, respectively, and the number of downregulated DEPs was 4.52 and 3.43 times that of upregulated DEPs in these two comparisons, respectively (Figure 2B). Venn diagrams were constructed and showed that the WT24h vs. WT0h and ZmPDI24h vs. ZmPDI0h comparisons had 4,069 (56.50%) DEGs in common, while only 73 (13.27%) common DEPs were common (Figures 2C,D).
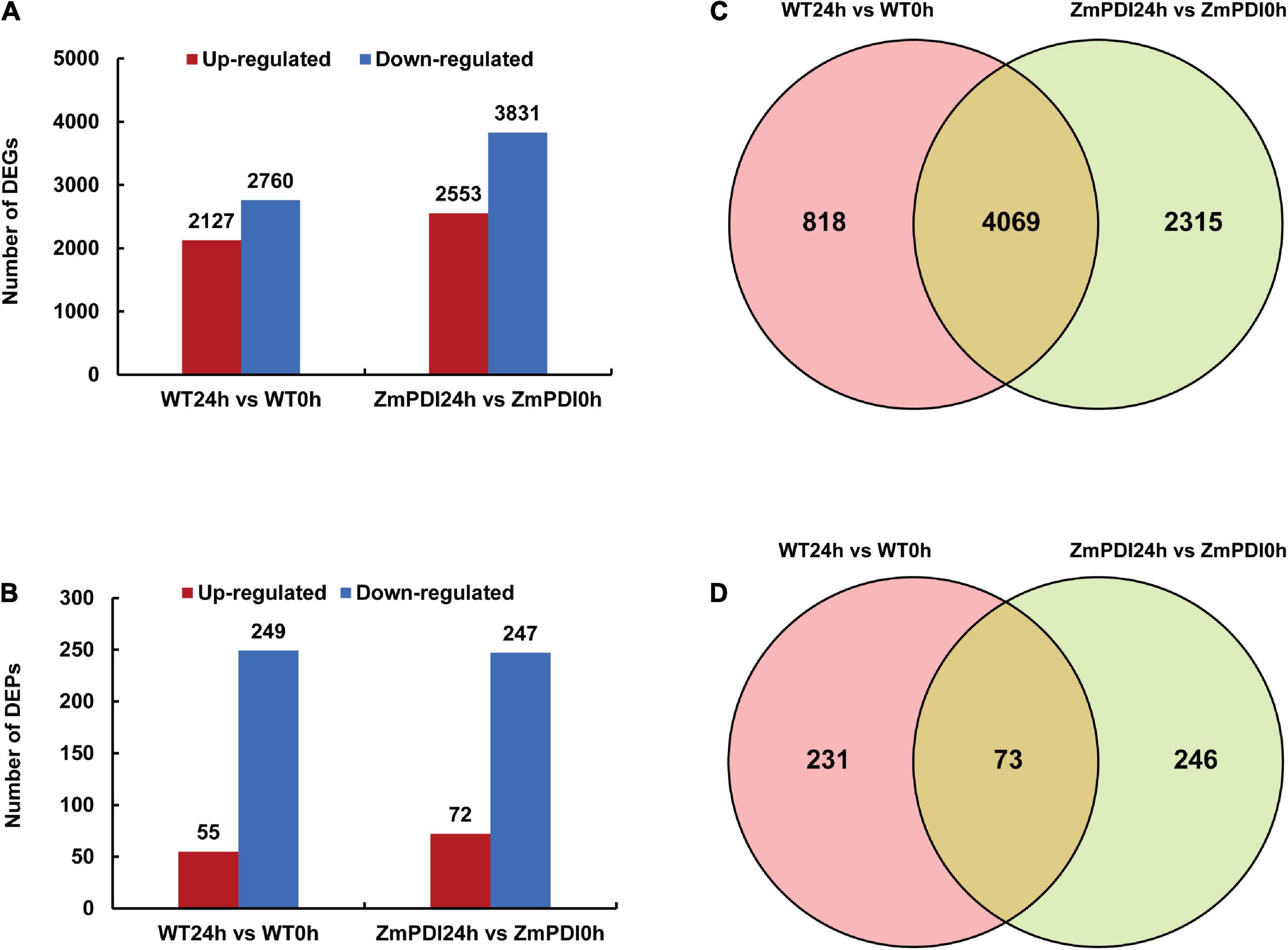
Figure 2. Salt tolerance-related transcriptome and proteome expression profiles of OX-ZmPDI transgenic and WT plants of Z. matrella. (A) The number of up- and downregulated DEGs in OX-ZmPDI transgenic (ZmPDI24h vs. ZmPDI0h) and wild-type plants (WT24h vs. WT0h). (B) The number of up- and downregulated DEPs in OX-ZmPDI transgenic (ZmPDI24h vs. ZmPDI0h) and wild-type plants (WT24h vs. WT0h). (C) Venn diagram of the number of DEGs in the OX-ZmPDI transgenic and wild-type plants after salt treatment. (D) Venn diagram of the number of DEPs in the OX-ZmPDI transgenic and wild-type plants after salt treatment.
Gene ontology and Kyoto Encyclopedia of Genes and Genomes pathway analysis of differentially expressed genes and differentially expressed proteins
To compare the differences in salt tolerance regulation mechanisms between wild-type and OX-ZmPDI transgenic plants, the functional characterization of DEGs and DEPs was classified by GO enrichment analysis. For RNA-seq, the top 20 enriched GO terms were selected in the WT24h vs. WT0h and ZmPDI24h vs. ZmPDI0h comparisons (Supplementary Table 3). A total of 13 GO terms were shared by both the WT24h vs. WT0h and ZmPDI24h vs. ZmPDI0h comparisons, while each comparison had seven unique GO terms (Figures 3A,B). In the ZmPDI24h vs. ZmPDI0h comparison, the unique GO terms included “cytoskeletal protein binding,” “polysaccharide metabolic process,” “tubulin binding,” “plant-type cell wall organization or biogenesis,” “external encapsulating structure organization,” “cytoskeletal part” and “response to abiotic stimulus,” and multiple terms were mainly related to the cytoskeleton (Figure 3B). Furthermore, except for “response to abiotic stimulus,” these unique GO terms in the ZmPDI24h vs. ZmPDI0h comparison had more downregulated DEGs (Figure 3B).
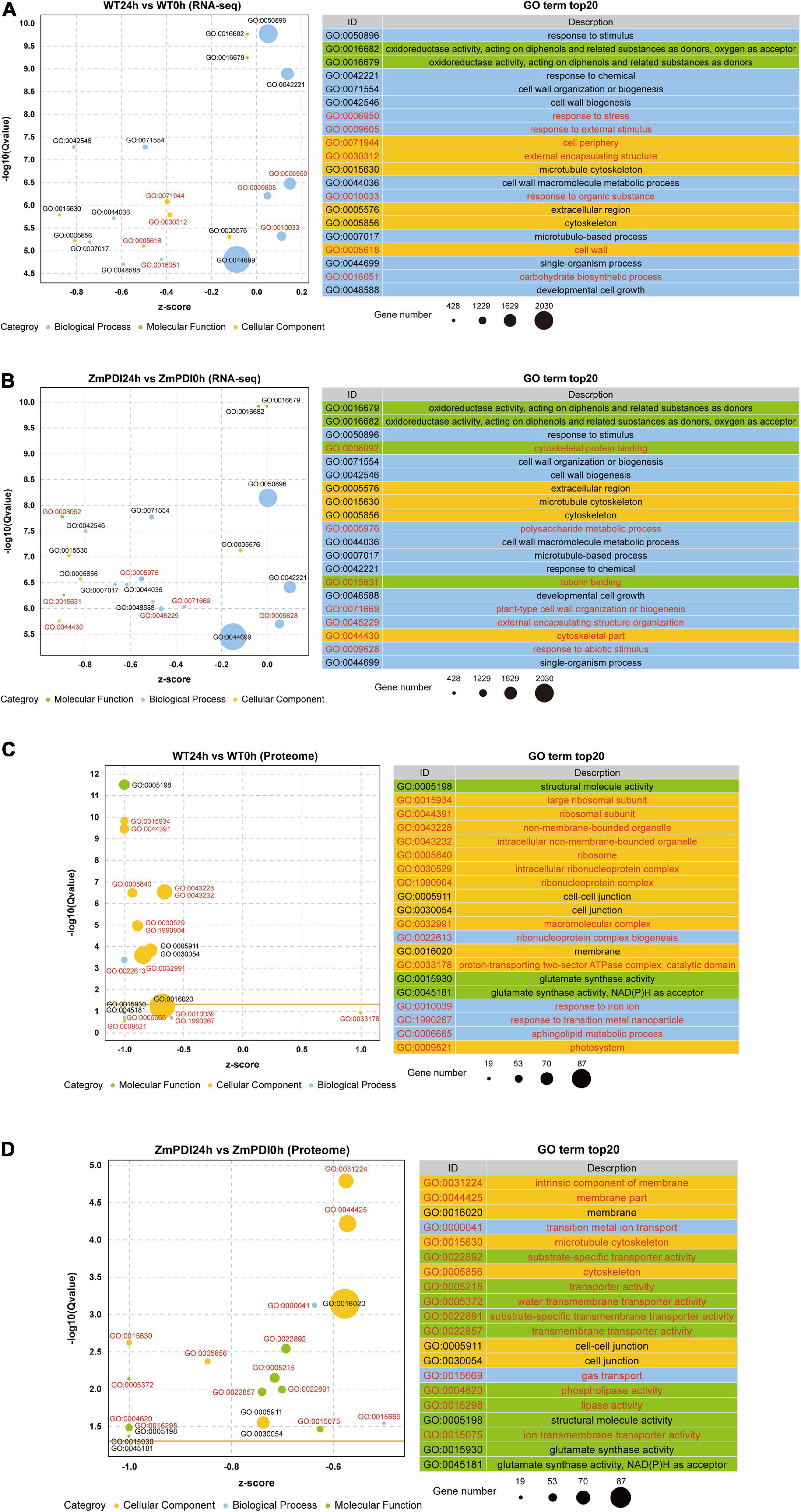
Figure 3. Top 20 enriched GO terms of DEGs and DEPs in OX-ZmPDI transgenic and WT plants of Z. matrella. (A) Top 20 enriched GO terms of DEGs in the WT24h vs. WT0h comparison. (B) Top 20 enriched GO terms of DEGs in the ZmPDI24h vs. ZmPDI0h comparison. (C) Top 20 enriched GO terms of DEPs in the WT24h vs. WT0h comparison. (D) Top 20 enriched GO terms of DEPs in the ZmPDI24h vs. ZmPDI0h comparison. The z-score value represents the ratio of the difference between the numbers of upregulated and downregulated genes to the total number of differentially expressed genes. The yellow line represents the threshold of Q-value = 0.05.
For proteome sequencing, the top 20 enriched GO terms were selected in the WT24h vs. WT0h and ZmPDI24h vs. ZmPDI0h comparisons, and only six GO terms were shared by both comparisons (Figures 3C,D and Supplementary Table 4). The WT24h vs. WT0h and ZmPDI24h vs. ZmPDI0h comparisons had 14 unique GO terms, and eight unique GO terms in the ZmPDI24h vs. ZmPDI0h comparison were related to transport or transport activity, including “transition metal ion transport,” “substrate-specific transporter activity,” “transporter activity,” “water transmembrane transporter activity,” “substrate-specific transmembrane transporter activity,” “transmembrane transporter activity,” “gas transport” and “ion transmembrane transporter activity” (Figures 3C,D). Comparing the top 20 enriched GO terms in the ZmPDI24h vs. ZmPDI0h comparison between RNA-seq and proteome sequencing, “microtubule cytoskeleton” and “cytoskeleton” terms were shared, and the DEGs in these two terms were mainly downregulated genes (Figures 3B,D).
The KEGG pathway analysis of DEGs for RNA-seq showed that 15 pathways were shared by both the WT24h vs. WT0h and ZmPDI24h vs. ZmPDI0h comparisons, and each comparison had five unique pathways (Figures 4A,B and Supplementary Table 5). In the ZmPDI24h vs. ZmPDI0h comparison, the five unique pathways included “Glycerolipid metabolism,” “Tyrosine metabolism,” “Starch and sucrose metabolism,” “Glyoxylate and dicarboxylate metabolism” and “Zeatin biosynthesis” (Figure 4B). Except for “starch and sucrose metabolism,” the other four unique pathways had more upregulated DEGs (Figure 4B). KEGG pathway analysis of DEPs for proteome sequencing showed that the top 20 pathways were selected in the WT24h vs. WT0h and ZmPDI24h vs. ZmPDI0h comparisons, and each comparison had nine unique pathways (Figures 4C,D and Supplementary Table 6). In the ZmPDI24h vs. ZmPDI0h comparison, multiple unique pathways were related to proteins, including “Protein processing in endoplasmic reticulum,” “Protein export” and “Ubiquitin mediated proteolysis,” and DEPs in these pathways were all downregulated (Figure 4D). Comparing the pathways in the ZmPDI24h vs. ZmPDI0h comparison, “phenylpropanoid biosynthesis,” “galactose metabolism,” “glutathione metabolism,” “nitrogen metabolism” and “phagosome” were shared between RNA-seq and proteome sequencing (Figures 4B,D).
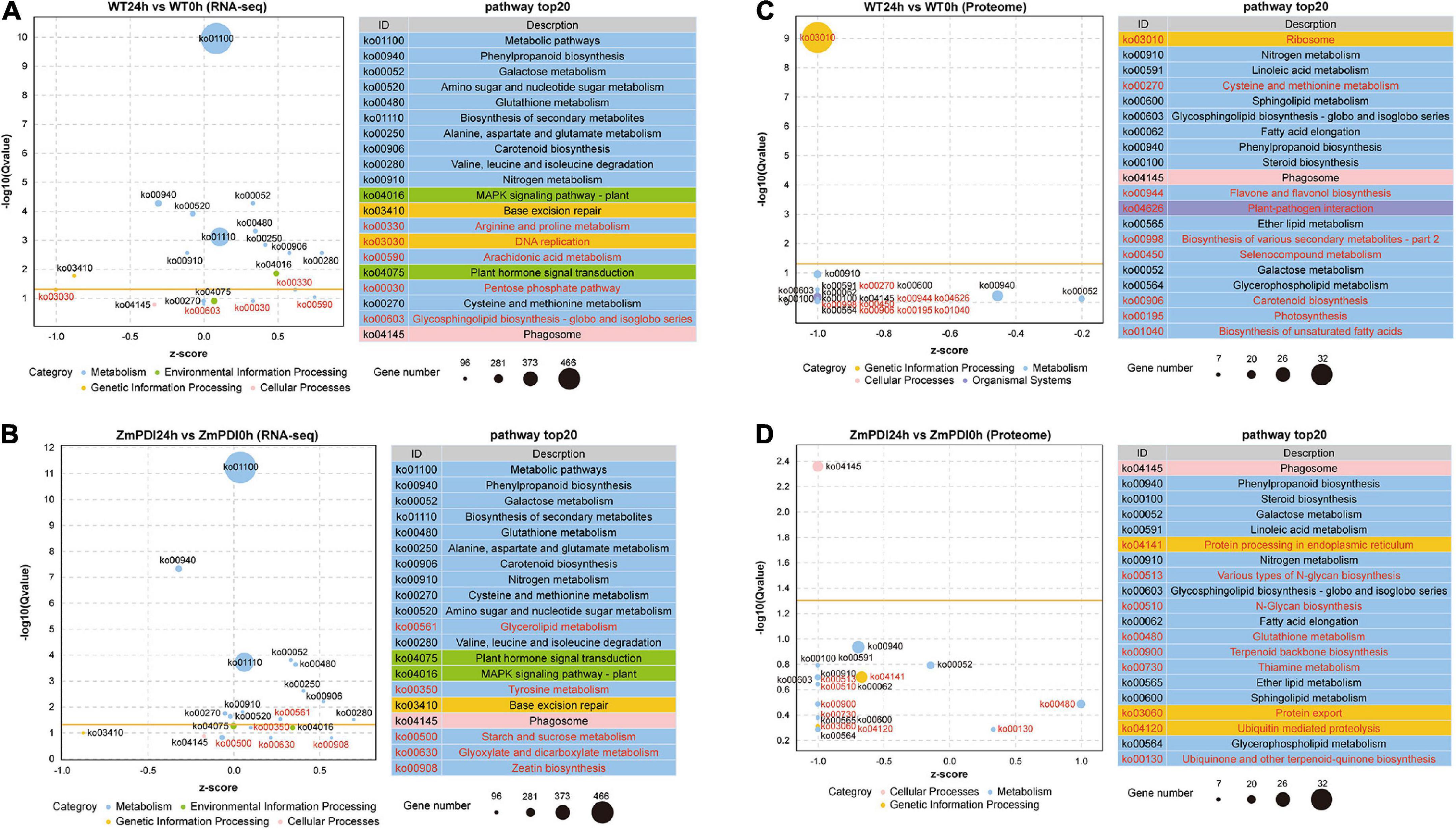
Figure 4. Top 20 enriched GO terms of DEGs and DEPs in OX-ZmPDI transgenic and WT plants of Z. matrella. (A) Top 20 enriched KEGG pathways of DEGs in the WT24h vs. WT0h comparison. (B) Top 20 enriched KEGG pathways of DEGs in the ZmPDI24h vs. ZmPDI0h comparison. (C) Top 20 enriched KEGG pathways of DEPs in the WT24h vs. WT0h comparison. (D) Top 20 enriched KEGG pathways of DEPs in the ZmPDI24h vs. ZmPDI0h comparison. The z-score value represents the ratio of the difference between the numbers of upregulated and downregulated genes to the total number of differentially expressed genes. The yellow line represents the threshold of Q-value = 0.05.
Transcriptome and proteome association analysis
To screen the DEGs with the same expression changes in the transcriptome and proteome, we performed an association analysis. In the WT24h vs. WT0h comparison, assessment of the number of genes revealed that there were 104 DEGs upregulated in the transcriptome and proteome, including 78 DEGs that did not meet the P-value threshold for significance (Supplementary Table 7). Meanwhile, 276 DEGs were downregulated in the transcriptome and proteome, including 240 DEGs that did not meet the P-value threshold (Supplementary Table 7). In the ZmPDI24h vs. ZmPDI0h comparison, the analysis of the number of genes revealed that there were 125 DEGs upregulated in the transcriptome and proteome, with 97 DEGs not meeting the P-value threshold (Supplementary Table 7). At the same time, 342 DEGs (including 285 DEGs that did not meet the P-value) were downregulated in the transcriptome and proteome (Supplementary Table 7).
The nine quadrant diagrams showed that upregulated and downregulated DEGs in the transcriptome and proteome were in quadrants 3 and 7, respectively, and the DEGs meeting the P-value are marked by red dots (Supplementary Figures 2C,D). Then, GO enrichment and KEGG pathway analyses were performed with the DEGs that met the P-value in quadrants 3 and 7. The GO enrichment analysis showed that the “cytoskeleton” and “microtubule cytoskeleton” terms were also more significantly enriched in the ZmPDI24h vs. ZmPDI0h comparison than in the WT24h vs. WT0h comparison (Supplementary Figures 3A,B and Supplementary Table 8). Comparing the KEGG pathways in the WT24h vs. WT0h comparison, seven unique metabolic pathways were enriched in the ZmPDI24h vs. ZmPDI0h comparison, including “ether lipid metabolism,” “stilbenoid, diarylheptanoid and gingerol biosynthesis,” “glycerophospholipid metabolism,” “cutin, suberine and wax biosynthesis,” “ubiquinone and other terpenoid-quinone biosynthesis,” “pentose phosphate pathway” and “glycolysis/gluconeogenesis” (Supplementary Figures 3C,D and Supplementary Table 9).
Differentially expressed gene identification and verification
In total, 119 DEGs that met the P-value threshold in quadrants 3 and 7 were selected from the WT24h vs. WT0h and ZmPDI24h vs. ZmPDI0h comparisons (Supplementary Figure 4). Most of these DEGs had similar expression trends between the WT24h vs. WT0h and ZmPDI24h vs. ZmPDI0h comparisons, and the protein levels of most DEGs were consistent with their RNA levels (Supplementary Figure 4). The number of DEGs in the OX-ZmPDI transgenic plants was very low compared with the wild-type plants either at the time point of salt treatment at 0h or 24h (Supplementary Figure 2C), which indicating that the degree of change in gene expression induced by salt stress in wild-type and OX-ZmPDI transgenic plants may be more important. Therefore, we set a selected condition of |log2[FC(ZmPDI24h/ZmPDI0h)/FC(WT24h/WT0h)]| > 1 and screened 36 DEGs significantly different between WT24h vs. WT0h and ZmPDI24h vs. ZmPDI0h comparisons at the RNA or proteome levels (Table 1).
Previous GO and KEGG pathway analyses in quadrants 3 and 7 showed that “cytoskeleton,” “microtubule cytoskeleton,” “ether lipid metabolism,” “stilbenoid, diarylheptanoid and gingerol biosynthesis,” “glycerophospholipid metabolism,” “cutin, suberine and wax biosynthesis,” “ubiquinone and other terpenoid-quinone biosynthesis,” “pentose phosphate pathway” and “glycolysis/gluconeogenesis” are significantly enriched in the OX-ZmPDI transgenic plants (Supplementary Figure 3). According to these GO terms and KEGG pathways, five important DEGs (TUBB2, PXG4, PLDα2, PFK4, and 4CL1) were selected from 36 DEGs, and they belonged to “cytoskeleton,” “cutin, suberine and wax biosynthesis,” “glycerophospholipid metabolism/ether lipid metabolism,” “pentose phosphate pathway/glycolysis/gluconeogenesis” and “ubiquinone and other terpenoid-quinone biosynthesis” respectively (Figure 5). Comparing to wild-type plants, the expression and protein levels of TUBB2, PXG4, PLDα2, and 4CL1 were downregulated and the levels of PFK4 were upregulated in the OX-ZmPDI transgenic plants under salt stress (Figure 5). These results indicated that the ZmPDI gene may enhance the salt tolerance of zoysiagrass by affecting the expression level or protein level of these genes. To confirm the reliability of RNA-seq, 12 genes selected from 36 DEGs (Table 1) were validated using qRT–PCR, and the results were largely consistent with the RNA-seq data (Supplementary Figure 5).
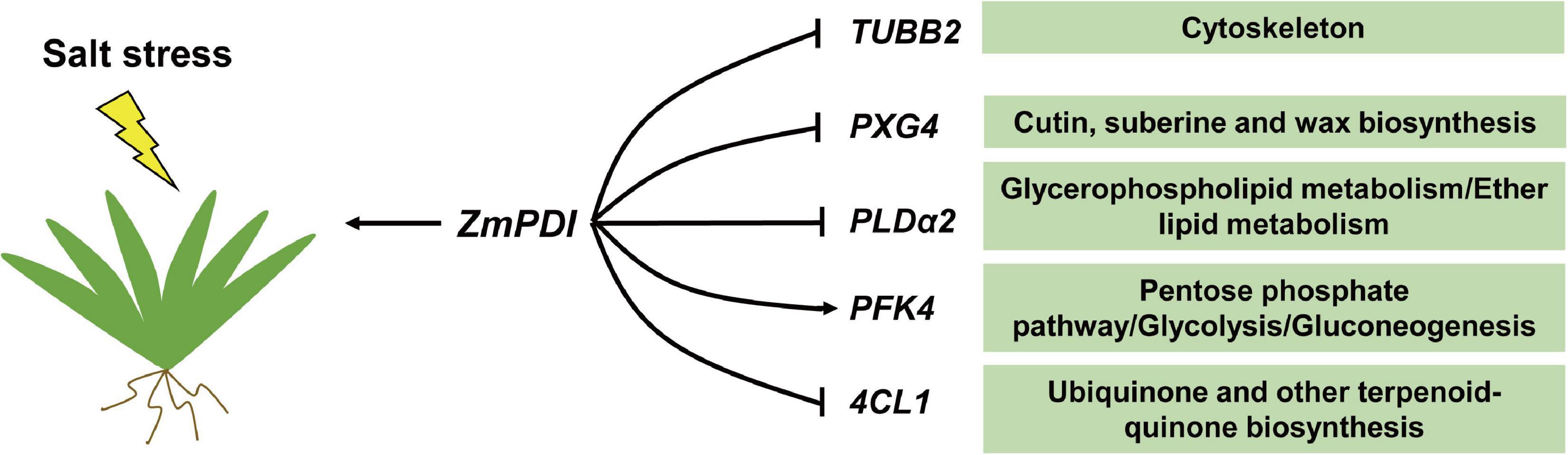
Figure 5. A predictive regulatory model of ZmPDI enhancing salt tolerance in Z. matrella. The contents in the green boxes are the GO term or KEGG pathways of the genes on the left belong.
Discussion
Overexpression of ZmPDI can enhance the salt tolerance of Zoysia matrella
Previous studies have shown that heterologous expression of ZmPDI enhances the salt tolerance of yeast (Chen et al., 2015b), but whether it has the same function in Z. matrella remains to be verified. In this study, homologous expression of ZmPDI in Z. matrella significantly improved salt tolerance while maintaining normal growth and aboveground and underground biomasses (Figures 1A–C). Under salinity stress, plant growth and development are inhibited mainly because of osmotic pressure disturbance, ionic imbalance and oxidative stress (Zhao et al., 2020). Plants regulate osmotic pressure mainly through two pathways, namely, the synthesis of organic osmolytes and improved absorption of inorganic ions (Zhao et al., 2020). Proline and sugars are organic osmolytes, and proline plays a dominant role in regulating osmotic pressure under salt stress (Zhao et al., 2020). In the OX-ZmPDI transgenic lines, the proline contents were significantly higher than those in the wild-type plants, while the soluble sugar contents were significantly lower (Figures 1D,E). These results indicated that OX-ZmPDI transgenic plants may synthesize more proline and activate glucose metabolism to adjust osmotic pressure under salinity stress.
Many enzymes involved in primary metabolism are present in the cytosolic compartment, and many of them are controlled by K+ (Zhao et al., 2020). However, Na+ is very similar to K+ and usually replaces K+ to involve those enzymatic reactions with much less efficiency (Zhao et al., 2020). Therefore, a high concentration of Na+ can disrupt metabolism and potentially kill the plant (Zhao et al., 2020). In OX-ZmPDI transgenic lines, the K+/Na+ ratios in roots were all significantly higher than those in wild-type plants after salt treatment (Figure 1F), indicating that ZmPDI can help plants maintain higher K+/Na+ ratios to resist Na+ damage. Reactive oxygen species (ROS) are rapidly induced by salinity stress and are mainly produced in the apoplast, chloroplasts, mitochondria and peroxisomes (Zhao et al., 2020). SOD can scavenge excess ROS to enhance the salt tolerance of plants (Zhao et al., 2020). Under salt treatment, overexpression of the ZmPDI gene significantly maintained normal SOD activity and chlorophyll content in Z. matrella (Figures 1H,I), indicating that normal SOD activity in chlorophyll may catalyze the scavenging of ROS and maintain the normal function of chloroplasts. These results proved that ZmPDI can enhance the salt tolerance of plants by maintaining osmotic pressure and ionic balance and reducing oxidative stress.
ZmPDI may enhance the salt tolerance of Zoysia matrella by regulating TUBB2, PXG4, PLDα2, PFK4, and 4CL1
To understand the difference in salt tolerance responses between wild-type and OX-ZmPDI transgenic plants, an association analysis of transcriptome and proteome sequencing was performed. The GO enrichment and KEGG pathway analyses of 119 DEGs that met the P-value threshold in quadrants 3 and 7 showed that ZmPDI may enhance the salt tolerance of Z. matrella in five aspects, namely, “cytoskeleton,” “cutin, suberine and wax biosynthesis,” “glycerophospholipid metabolism/ether lipid metabolism,” “pentose phosphate pathway/glycolysis/gluconeogenesis” and “ubiquinone and other terpenoid-quinone biosynthesis” (Figure 5). The plant cytoskeleton is mainly composed of microtubules (MTs), microfilaments (MFs) and MT/MF-interacting proteins, and its dynamic organizational changes can enhance plant tolerance through various intracellular activities, including cell morphogenesis and cell signal transduction (Yang and Guo, 2018; Zhao et al., 2021). Salt stress induces the depolymerization and reorganization of MTs, and its destabilization can enhance the salt tolerance of plants (Zhao et al., 2021). However, MF depolymerization is related to cell death under long-term salt stress, and its stabilization can increase the ability of plants to withstand salt stress (Yang and Guo, 2018; Zhao et al., 2021). A cytoskeletal structural component gene, beta-2-tubulin (TUBB2, Zjn_sc00009.1.g09140.1.sm.mk), was screened as a DEG and downregulated in OX-ZmPDI transgenic plants at both the transcriptome and proteome levels (Table 1). Tubulin is the major constituent of the cytoskeleton, which forms networks of microtubules (Chiolerio et al., 2020). These results indicated that ZmPDI may improve the salt tolerance of zoysiagrass by downregulating TUBB2 levels to affect the destabilization of MTs.
Epoxygenated fatty acids are components of cutin and suberin and act as defense molecules (Blee et al., 2012). The caleosin-type peroxygenases catalyze the epoxidation of unsaturated fatty acids in the presence of hydroperoxides to form epoxygenated fatty acids (Blee et al., 2012). Peroxygenase 4 (PXG4, Zjn_sc00093.1.g00470.1.sm.mkhc) is a class II caleosin gene that encodes a calcium-binding peroxygenase involved in the degradation of storage lipids (Blee et al., 2012). In Arabidopsis, another name of the PXG4 gene is AtCLO4, and previous studies have confirmed that PXG4 participates in salt tolerance by acting as a negative regulator of abscisic acid (ABA) (Kim et al., 2011; Blee et al., 2012). During salt stress, the clo4 mutants perform significantly less inhibition of lateral root formation (Rafeh, 2016). After salt treatments, the “cutin, suberine and wax biosynthesis” and PXG4 genes were significantly enriched and screened in OX-ZmPDI transgenic plants (Figure 5). Compared with wild-type plants, the transcript and protein levels of PXG4 in OX-ZmPDI transgenic plants were all decreased (Table 1). These results indicated that ZmPDI may inhibit PXG4 expression to decrease this protein level, thereby maintaining lateral root formation to improve the salt tolerance of zoysiagrass.
Phospholipase D (PLD) is a phosphatidyl choline-hydrolyzing enzyme that can generate the lipid second messenger phosphatidic acid (PA) by hydrolyzing membrane phospholipids, and the expression of multiple PLD genes is increased by exposure to various stresses (Bargmann and Munnik, 2006; Ji et al., 2017). PLDs are involved in multiple cellular processes, including membrane composition and microtubule and cytoskeletal dynamics (Faraudo and Travesset, 2007; Zhang et al., 2017). The expression levels of some PLD genes are induced by salt stress, and pldα1, pldα3, and pldδ mutants show salt sensitivity (Hunter et al., 2019). Among them, the molecular mechanism by which PLDα1 responds to salt stress has been studied in depth. PLDα1 interacts with mitogen-activated protein kinases 3 (MPK3) to enhance salt tolerance and ABA signaling (Vadovič et al., 2019). In addition, Cys-rich receptor-like kinase 2 (CRK2) acts as the downstream target protein of PLDα1 and enhances salt tolerance at the germination stage in Arabidopsis (Hunter et al., 2019). Previous studies have shown that PLDα2 is highly induced by salt and drought stress in rice (Li et al., 2007). However, the transcript and protein levels of PLDα2 in OX-ZmPDI transgenic and wild-type plants all decreased after salt treatments (Table 1). The protein alignment of PLDα2 genes in Z. matrella and Oryza sativa showed that the amino acid sequences of ZmPLDα2 and OsPLDα2 have an obviously difference (Supplementary Figure 6A). These results indicated that PLDα2 may play a different role in regulating the salt tolerance of zoysiagrass.
In the plant glycolytic pathway, ATP-dependent 6-phosphofructokinase (PFK) catalyzes the phosphorylation of fructose 6-phosphate (F6P), transforming it to fructose-1,6-bisphosphate (F16BP) (Zhong et al., 2016). In previous studies, the expression levels of PFK were significantly induced by salt stress in rice, cucumber and tomato (Zhong et al., 2016; Yuenyong et al., 2018; Feng et al., 2019). Soluble sugar is an organic osmolyte that can balance the vacuole solute potential, but the accumulation of sugar in leaves can inhibit photosynthesis (Zhong et al., 2016). In our studies, compared with wild-type plants, the transcript and protein levels of PFK4 in OX-ZmPDI transgenic plants were all increased (Table 1). The soluble sugar contents were significantly lower and the chlorophyll contents maintained significant high levels in OX-ZmPDI transgenic plant leaves (Figures 1E,H). These results indicated that ZmPDI may increase PFK4 expression to accelerate the conversion of F6P into F16BP, thereby enhancing the glycolysis pathway to maintain normal photosynthetic efficiency and chlorophyll content in zoysiagrass.
4-Coumarate-CoA ligase (4CL) is an important branch point involved in phenylpropanoid metabolism that regulates the biosynthesis of flavonoids, lignin and other phenolic secondary metabolites and plays important roles in plant physiology and biotic and abiotic stresses (Lavhale et al., 2018; Chen et al., 2020; Wang et al., 2022). 4CL genes emerge in response to salt stress in a variety of plants. In desert poplars, the expression of Pp4CL2, Pp4CL11, and Pp4CL12 is increased significantly in response to salt stress compared with salt-sensitive poplar (Zhang et al., 2015). In Arabidopsis, higher expression levels of lignin biosynthesis-related genes, including 4CL1 and 4CL2, are detected in salt-adapted cells with increased lignin content and thickened cell walls relative to normal cells (Chun et al., 2019). In Sophora alopecuroides, transcriptomic analysis revealed that Sa4CL, which is involved in lignin synthesis, is significantly upregulated under salt stress (Zhu et al., 2021). In our study, a 4CL1 gene was screened between wild-type and OX-ZmPDI transgenic plants (Table 1). However, the 4CL1 gene was downregulated after salt treatment in transcriptome sequencing, and its downregulation was more obvious in OX-ZmPDI transgenic plants (Table 1), indicating that ZmPDI may influence the expression of 4CL1 to regulate the salt tolerance of zoysiagrass. The protein alignment of 4CL1 genes in Z. matrella and Arabidpsis showed that the amino acid sequences of Zm4CL1 and At4CL1 have an obviously difference (Supplementary Figure 6B). Therefore, the function of 4CL1 in zoysiagrass may be different from that in other plants.
Data availability statement
The datasets presented in this study can be found in online repositories. The names of the repository/repositories and accession number(s) can be found in the article/Supplementary material.
Author contributions
QM and KW performed the evaluation experiments. JW, QM, and KW performed the transcriptomic and proteome analyses and verification experiments. JL and JW designed the experiment. XL, PW, HG, JC, and JZ supervised the project. JW and QM participated in writing the manuscript. All authors contributed to the article and approved the submitted version.
Funding
This work was funded by the National Natural Science Foundation of China (Grant Nos. 31672195, 31901383, and 32002081) and the Natural Science Foundation of Jiangsu Province, China (Grant Nos. BK20190274 and BK20200285).
Conflict of interest
The authors declare that the research was conducted in the absence of any commercial or financial relationships that could be construed as a potential conflict of interest.
Publisher’s note
All claims expressed in this article are solely those of the authors and do not necessarily represent those of their affiliated organizations, or those of the publisher, the editors and the reviewers. Any product that may be evaluated in this article, or claim that may be made by its manufacturer, is not guaranteed or endorsed by the publisher.
Supplementary material
The Supplementary Material for this article can be found online at: https://www.frontiersin.org/articles/10.3389/fpls.2022.970651/full#supplementary-material
Footnotes
References
Ali Khan, H., and Mutus, B. (2014). Protein disulfide isomerase a multifunctional protein with multiple physiological roles. Front. Chem. 2:70. doi: 10.3389/fchem.2014.00070
Bargmann, B. O., and Munnik, T. (2006). The role of phospholipase D in plant stress responses. Curr. Opin. Plant Biol. 9, 515–522. doi: 10.1016/j.pbi.2006.07.011
Blee, E., Flenet, M., Boachon, B., and Fauconnier, M. L. (2012). A non-canonical caleosin from A rabidopsis efficiently epoxidizes physiological unsaturated fatty acids with complete stereoselectivity. FEBS J. 279, 3981–3995. doi: 10.1111/j.1742-4658.2012.08757.x
Chen, J., Yan, J., Qian, Y., Jiang, Y., Zhang, T., Guo, H., et al. (2009). Growth responses and ion regulation of four warm season turfgrasses to long-term salinity stress. Sci. Hortic. 122, 620–625. doi: 10.1016/j.scienta.2009.07.004
Chen, X., Su, W., Zhang, H., Zhan, Y., and Zeng, F. (2020). Fraxinus mandshurica 4-coumarate-CoA ligase 2 enhances drought and osmotic stress tolerance of tobacco by increasing coniferyl alcohol content. Plant Physiol. Bioch. 155, 697–708. doi: 10.1016/j.plaphy.2020.08.031
Chen, Y., Li, L., Zong, J., Chen, J., Guo, H., Guo, A., et al. (2015a). Heterologous expression of the halophyte Zoysia matrella H+-pyrophosphatase gene improved salt tolerance in Arabidopsis thaliana. Plant Physiol. Bioch. 91, 49–55. doi: 10.1016/j.plaphy.2015.04.004
Chen, Y., Zong, J., Tan, Z., Li, L., Hu, B., Chen, C., et al. (2015b). Systematic mining of salt-tolerant genes in halophyte-Zoysia matrella through cDNA expression library screening. Plant Physiol. Bioch. 89, 44–52. doi: 10.1016/j.plaphy.2015.02.007
Chiolerio, A., Draper, T. C., Mayne, R., and Adamatzky, A. (2020). On resistance switching and oscillations in tubulin microtubule droplets. J. Colloid Interf. Sci. 560, 589–595. doi: 10.1016/j.jcis.2019.10.065
Chun, H. J., Baek, D., Cho, H. M., Lee, S. H., Jin, B. J., Yun, D. J., et al. (2019). Lignin biosynthesis genes play critical roles in the adaptation of Arabidopsis plants to high-salt stress. Plant Signal Behav. 14:1625697. doi: 10.1080/15592324.2019.1625697
Fan, F., Zhang, Y., Wang, S., Han, Y., Wang, L., and Lu, D. (2018). Characterization of the oxidative protein folding activity of a unique plant oxidoreductase, Arabidopsis protein disulfide isomerase-11. Biochem. Bioph. Res. Co. 495, 1041–1047. doi: 10.1016/j.bbrc.2017.11.111
Fang, C., Ye, Z., Gai, T., Lu, K., Dai, F., Lu, C., et al. (2021). DIA-based proteome reveals the involvement of cuticular proteins and lipids in the wing structure construction in the silkworm. J. Proteomics 238:104155. doi: 10.1016/j.jprot.2021.104155
Faraudo, J., and Travesset, A. (2007). Phosphatidic acid domains in membranes: Effect of divalent counterions. Biophys. J. 92, 2806–2818. doi: 10.1529/biophysj.106.092015
Feng, Y., Chen, X., He, Y., Kou, X., and Xue, Z. (2019). Effects of exogenous trehalose on the metabolism of sugar and abscisic acid in tomato seedlings under salt stress. Trans. Tianjin Univ. 25, 451–471. doi: 10.1007/s12209-019-00214-x
Ge, Y. X., Norton, T., and Wang, Z. Y. (2006). Transgenic zoysiagrass (Zoysia japonica) plants obtained by Agrobacterium-mediated transformation. Plant Cell Rep. 25, 792–798. doi: 10.1007/s00299-006-0123-8
Hunter, K., Kimura, S., Rokka, A., Tran, H. C., Toyota, M., Kukkonen, J. P., et al. (2019). CRK2 enhances salt tolerance by regulating callose deposition in connection with PLDα1. Plant Physiol. 180, 2004–2021. doi: 10.1104/pp.19.00560
Ji, T., Li, S., Huang, M., Di, Q., Wang, X., Wei, M., et al. (2017). Overexpression of cucumber phospholipase D alpha gene (CsPLDα) in tobacco enhanced salinity stress tolerance by regulating Na+-K+ balance and lipid peroxidation. Front. Plant Sci. 8:499. doi: 10.3389/fpls.2017.00499
Jiang, Q., Chen, J., Zong, J., Li, S., Chu, X., Guo, H., et al. (2013). Effect of phosphorus on Na+ and K+ concentrations and the growth of Zoysia matrella under salt stress. Acta Pratacult. Sin. 22, 162–168. doi: 10.11686/cyxb20130321
Kim, D., Langmead, B., and Salzberg, S. L. (2015). HISAT: A fast spliced aligner with low memory requirements. Nat. Methods 12, 357–360. doi: 10.1038/nmeth.3317
Kim, Y. Y., Jung, K. W., Yoo, K. S., Jeung, J. U., and Shin, J. S. (2011). A stress-responsive caleosin-like protein, AtCLO4, acts as a negative regulator of ABA responses in Arabidopsis. Plant Cell Physiol. 52, 874–884. doi: 10.1093/pcp/pcr039
Lavhale, S. G., Kalunke, R. M., and Giri, A. P. (2018). Structural, functional and evolutionary diversity of 4-coumarate-CoA ligase in plants. Planta 248, 1063–1078. doi: 10.1007/s00425-018-2965-z
Li, G., Lin, F., and Xue, H. W. (2007). Genome-wide analysis of the phospholipase D family in Oryza sativa and functional characterization of PLDβ1 in seed germination. Cell Res. 17, 881–894. doi: 10.1038/cr.2007.77
Li, S., Chen, J., Guo, H., Zong, J., Zhang, F., Chu, X., et al. (2012). Salinity tolerance evaluation of Zoysia turfgrass germplasm. Acta Pratacult. Sin. 21, 43–51.
Love, M. I., Huber, W., and Anders, S. (2014). Moderated estimation of fold change and dispersion for RNA-seq data with DESeq2. Genome Biol. 15, 1–21. doi: 10.1186/s13059-014-0550-8
Marcum, K. B., and Murdoch, C. L. (1994). Salinity tolerance mechanisms of six C4 turfgrasses. J. Am. Soc. Hortic. Sci. 119, 779–784. doi: 10.21273/JASHS.119.4.779
Mortazavi, A., Williams, B. A., McCue, K., Schaeffer, L., and Wold, B. (2008). Mapping and quantifying mammalian transcriptomes by RNA-Seq. Nat. Methods 5, 621–628. doi: 10.1038/nmeth.1226
Okumura, M., Noi, K., and Inaba, K. (2021). Visualization of structural dynamics of protein disulfide isomerase enzymes in catalysis of oxidative folding and reductive unfolding. Curr. Opin. Struc. Biol. 66, 49–57. doi: 10.1016/j.sbi.2020.10.004
Pertea, M., Pertea, G. M., Antonescu, C. M., Chang, T. C., Mendell, J. T., and Salzberg, S. L. (2015). StringTie enables improved reconstruction of a transcriptome from RNA-seq reads. Nat. Biotechnol. 33, 290–295. doi: 10.1038/nbt.3122
Rafeh, R. (2016). AtCLO4, a stress responsive calcium binding protein, interacts with the heterotrimeric Gα subunit in Arabidopsis thaliana. Doctoral dissertation. Montreal: Concordia University.
Robinson, M. D., McCarthy, D. J., and Smyth, G. K. (2010). edgeR: A Bioconductor package for differential expression analysis of digital gene expression data. Bioinformatics 26, 139–140. doi: 10.1093/bioinformatics/btp616
Tanaka, H., Hirakawa, H., Muguerza, M., Hashiguchi, M., Tabata, S., Akashi, R., et al. (2016a). The complete chloroplast genome sequence of Zoysia matrella (L.) merr. Crop Sci. 56, 1206–1212. doi: 10.2135/cropsci2015.08.0517
Tanaka, H., Hirakawa, H., Kosugi, S., Nakayama, S., Ono, A., Watanabe, A., et al. (2016b). Sequencing and comparative analyses of the genomes of zoysiagrasses. DNA Res. 23, 171–180. doi: 10.1093/dnares/dsw006
Tominaga, J., Nakahara, Y., Horikawa, D., Tanaka, A., Kondo, M., Kamei, Y., et al. (2018). Overexpression of the protein disulfide isomerase AtCYO1 in chloroplasts slows dark-induced senescence in Arabidopsis. BMC Plant Biol. 18:80. doi: 10.1186/s12870-018-1294-5
Vadovič, P., Šamajová, O., Takáč, T., Novák, D., Zapletalová, V., Colcombet, J., et al. (2019). Biochemical and genetic interactions of phospholipase D alpha 1 and mitogen-activated protein kinase 3 affect Arabidopsis stress response. Front. Plant Sci. 10:275. doi: 10.3389/fpls.2019.00275
Wang, J., An, C., Guo, H., Yang, X., Chen, J., Zong, J., et al. (2020). Physiological and transcriptomic analyses reveal the mechanisms underlying the salt tolerance of Zoysia japonica Steud. BMC Plant Biol. 20:114. doi: 10.1186/s12870-020-02330-6
Wang, K., Wang, Y., Qu, A., Wang, R., Guo, H., Li, X., et al. (2020). Establishment of genetic transformation system for Zoysia matrella. Chin. J. Trop. Crops 41:1566. doi: 10.3969/j.issn.1000-2561.2020.08.009
Wang, Y., Guo, L., Zhao, Y., Zhao, X., and Yuan, Z. (2022). Systematic analysis and expression profiles of the 4-Coumarate: CoA ligase (4CL) gene family in pomegranate (Punica granatum L.). Int. J. Mol. Sci. 23:3509. doi: 10.3390/ijms23073509
Xie, Q., Niu, J., Xu, X., Xu, L., Zhang, Y., Fan, B., et al. (2015). De novo assembly of the Japanese lawngrass (Zoysia japonica Steud.) root transcriptome and identification of candidate unigenes related to early responses under salt stress. Front. Plant Sci. 6:610. doi: 10.3389/fpls.2015.00610
Yamamoto, A., Hashiguchi, M., Akune, R., Masumoto, T., Muguerza, M., Saeki, Y., et al. (2016). The relationship between salt gland density and sodium accumulation/secretion in a wide selection from three Zoysia species. Aust. J. Bot. 64, 277–284. doi: 10.1071/BT15261
Yang, Y., and Guo, Y. (2018). Elucidating the molecular mechanisms mediating plant salt-stress responses. New Phytol. 217, 523–539. doi: 10.1111/nph.14920
Yuenyong, W., Chinpongpanich, A., Comai, L., Chadchawan, S., and Buaboocha, T. (2018). Downstream components of the calmodulin signaling pathway in the rice salt stress response revealed by transcriptome profiling and target identification. BMC Plant Biol. 18:1–23. doi: 10.1186/s12870-018-1538-4
Zhang, C. H., Ma, T., Luo, W. C., Xu, J. M., Liu, J. Q., and Wan, D. S. (2015). Identification of 4CL genes in desert poplars and their changes in expression in response to salt stress. Genes 6, 901–917. doi: 10.3390/genes6030901
Zhang, Q., Qu, Y., Wang, Q., Song, P., Wang, P., Jia, Q., et al. (2017). Arabidopsis phospholipase D alpha 1-derived phosphatidic acid regulates microtubule organization and cell development under microtubule-interacting drugs treatment. J. Plant Res. 130, 193–202. doi: 10.1007/s10265-016-0870-8
Zhang, Z., Liu, X., Li, R., Yuan, L., Dai, Y., and Wang, X. (2018). Identification and functional analysis of a protein disulfide isomerase (AtPDI1) in Arabidopsis thaliana. Front. Plant Sci. 9:913. doi: 10.3389/fpls.2018.00913
Zhao, C., Zhang, H., Song, C., Zhu, J. K., and Shabala, S. (2020). Mechanisms of plant responses and adaptation to soil salinity. Innovation 1:100017. doi: 10.1016/j.xinn.2020.100017
Zhao, S., Zhang, Q., Liu, M., Zhou, H., Ma, C., and Wang, P. (2021). Regulation of plant responses to salt stress. Int. J. Mol. Sci. 22:4609. doi: 10.3390/ijms22094609
Zhong, M., Yuan, Y., Shu, S., Sun, J., Guo, S., Yuan, R., et al. (2016). Effects of exogenous putrescine on glycolysis and Krebs cycle metabolism in cucumber leaves subjected to salt stress. Plant Growth Regul. 79, 319–330. doi: 10.1007/s10725-015-0136-9
Keywords: Zoysia matrella, salt tolerance, PDI, RNA-seq, proteome
Citation: Ming Q, Wang K, Wang J, Liu J, Li X, Wei P, Guo H, Chen J and Zong J (2022) The combination of RNA-seq transcriptomics and data-independent acquisition proteomics reveals the mechanisms underlying enhanced salt tolerance by the ZmPDI gene in Zoysia matrella [L.] Merr. Front. Plant Sci. 13:970651. doi: 10.3389/fpls.2022.970651
Received: 16 June 2022; Accepted: 22 July 2022;
Published: 08 August 2022.
Edited by:
Kehua Wang, China Agricultural University, ChinaReviewed by:
Kun Zhang, Qingdao Agricultural University, ChinaJinhui Chen, Hainan University, China
Copyright © 2022 Ming, Wang, Wang, Liu, Li, Wei, Guo, Chen and Zong. This is an open-access article distributed under the terms of the Creative Commons Attribution License (CC BY). The use, distribution or reproduction in other forums is permitted, provided the original author(s) and the copyright owner(s) are credited and that the original publication in this journal is cited, in accordance with accepted academic practice. No use, distribution or reproduction is permitted which does not comply with these terms.
*Correspondence: Jingjing Wang, c2hlcmxvY2s0N0AxMjYuY29t
†These authors have contributed equally to this work