- Department of Ecological and Biological Sciences, University of Tuscia, Viterbo, Italy
Plant growth and response to environmental cues are largely driven by hormones. Salicylic acid (SA)- and jasmonic acid (JA)-mediated defenses have been shown to be effective against different types of attackers. SA-mediated defense is mainly effective against biotrophic pathogens and phloem-feeding insects, whereas JA-mediated defense is effective against necrotrophic pathogens and tissue-damaging insects. Cytokinins (CKs) are classic growth hormones that have also emerged as plant immunity modulators. Evidence pointed out that CKs contribute to the defense responses mediated by SA and JA, acting as hormone modulators of the SA/JA signaling backbone. Recently, we identified in Arabidopsis a type-B response regulator 11 (ARR 11) involved in cytokinin-mediated responses as a novel regulator of the SA/JA cross-talk. Here we investigated plant fitness and resistance against the fungal necrotrophic pathogen Botrytis cinerea in Arabidopsis wild-type Col-8 and defective arr11 mutant following SA, JA, CK single or combined treatment. Our results demonstrated that the CK and SA/JA/CK combination has a positive outcome on plant fitness in both Arabidopsis Col-8 and arr11 mutant,. The triple hormone treatment is efficient in increasing resistance to B. cinerea in Col-8 and this effect is stronger in arr11 mutant. The results will provide not only new background knowledge, corroborating the role of ARR11 in plant-defense related processes, but also new potential opportunities for alternative ways of protecting plants from fungal diseases.
Introduction
Plant hormones are key players in plant immunity. The type of attacker can determine what type of hormone accumulates in the plant, and each hormone regulates its own core pathway in the immune network (Aerts et al., 2020; Aerts et al., 2022). The defense pathways regulated by jasmonic acid (JA) and salicylic acid (SA), which form the backbone of the hormone‐regulated part of the immune system, are among the most studied (Wasternack and Song, 2017; Zhang and Li, 2019; Aerts et al., 2020). The JA pathway can be subdivided into two branches. The ERF branch is activated by necrotrophic pathogen infection while the MYC branch generally provides protection against chewing insects. The SA pathway is considered primarily directed against biotrophic pathogens (Pieterse et al., 2012). Additionally, other plant hormones, such as auxins, abscisic acid (ABA), cytokinins (CKs), gibberellins, and brassinosteroids, which have been thoroughly described to regulate plant development and growth, have emerged as key regulators of plant immunity (Pieterse et al., 2012). In particular, CKs are among the most important signaling molecules for regulating growth and development throughout the life of the plant and largely involved in cell division, growth and organogenesis, vascular differentiation, lateral root initiation, gravitropism and phototropism as well as fruit development (Osugi and Sakakibara, 2015; Wu et al., 2021). All plant hormonal pathways are linked to each other in a huge, complex, and largely still obscure network, to balance the response to developmental and environmental cues, as a cost-saving strategy (Pieterse et al., 2012; Thaler et al., 2012; Aerts et al., 2020). The classical example of cross-talk in defense regulation is that between the SA and JA pathways. The antagonism between these two pathways is the most studied and prevalent, although large-scale additive and synergistic interactions have been reported (Hickman et al., 2019; Aerts et al., 2020). The synergistic or antagonistic impact of CKs on SA- and JA-mediated defense responses has been reported in recent years (Zhang et al., 2022 and references therein). For instance, dose-dependent CKs application can modulate SA-mediated resistance to the biotrophic oomycete pathogen Hyaloperonospora arabidopsidis (Argueso et al., 2012). Moreover, the synergistic interaction between SA and CK has been shown to increase rice resistance to infection of the blast fungus Magnaporthe oryzae (Jiang et al., 2013). In addition, CKs have been reported to enhance JA-mediated resistance to the virulent necrotrophic fungus Alternaria brassicicola (Choi et al., 2010). To the best of our knowledge, beyond the combination CK/SA and CK/JA, the impact of SA/JA/CK combined treatment on pathogen resistance, as well as on growth, has not yet been investigated. Recently, from a GWA study performed in Arabidopsis, we identified ARR11 as a novel player involved in the effect of SA on JA pathway, using PDF1.2 gene as JA-marker. Additionally, in arr11 defective mutant we found altered resistance against the fungal necrotrophic pathogen Botrytis cinerea, compared to the wild-type Col-8 (Proietti et al., 2018). Furthermore, besides the involvement of ARR11 in SA/JA cross-talk, this protein has been shown to play a role in cross-communication between CK and ABA signaling (Huang et al., 2018). ARR11 (TAIR code: At1g16770) belongs to the family of type-B ARRs (Arabidopsis Response Regulators) which plays a pivotal role in the early transcriptional response of plants to cytokinins (Kushwah et al., 2011). The type-B ARRs are structurally related, each possessing a receiver domain phosphorylated on a conserved Asp residue, as well as a long C-terminal extension with a Myb-like DNA-binding domain (Imamura et al., 1999; Hosoda et al., 2002). The ability of the Myb-like domain to bind DNA has been demonstrated in several studies (Sakai et al., 2000; Hosoda et al., 2002) and multiple lines of evidence support a role of type-B ARRs as transcription factors (Liang et al., 2012; Tsai et al., 2012).
Here we investigated the involvement of ARR11 in SA/JA/CK-mediated responses in Arabidopsis. We demonstrated that SA/JA/CK triple treatment has a positive impact on plant fitness and resistance against B. cinerea in both Arabidopsis wild-type Col-8 and arr11 mutant. Nevertheless, in the latter, the combined hormone treatment is even more efficient in increasing resistance to B. cinerea suggesting ARR11 as a player in plant defense related processes.
Materials and methods
Plant material and growth conditions
A. thaliana T-DNA line in Col-8 background (arr11) was acquired from NASC (http://arabidopsis.info/) (SALK_006544C), as already described (Proietti et al., 2018). Seeds of the Arabidopsis arr11 and Col-8 were sown in cultivation containers filled with autoclaved river sand, supplied with half-strength Hoagland solution (Sigma, Steinheim, Germany). To reach high relative humidity for germination, the cultivation containers were enclosed in a tray with water and covered with a transparent lid. Seeds were incubated for 2 days at 4°C in the dark to ensure homogeneous germination, after which the tray was moved to a growth chamber with 8-h day/16-h night rhythm, a temperature of 21°C, and a light intensity of 100 μmol m-2 sec-1. After a week, the lids were slightly opened and gradually removed over a 2-day period. Ten-day-old seedlings were transplanted to individual pots containing autoclaved mixture of river sand and potting soil (1:1, v:v). Pots were supplied with water from the bottom three times per week. At the age of 3 weeks the plants were supplied once a week with half strength Hoagland solution.
Chemical treatments
Five-week-old A. thaliana plants (Col-8 and arr11 T-DNA line) were treated individually with SA (Sigma, Steinheim, Germany), MeJA (Serva, Brunschwig Chemie, Amsterdam, the Netherlands), CK (Sigma, Steinheim, Germany) or in combination, by dipping plants in a solution containing 0.015% (v:v) Silwet L77 (Van Meeuwen Chemicals BV, Weesp, the Netherlands) supplemented with either 1 mM SA or 100 μM MeJA or 15 μM CK or a combination of the three hormones at the same concentration. MeJA was diluted from a 1000-fold concentrated stock in 96% ethanol. The mock solution contained 0.015% Silwet L77 only. The 5th and 11th leaves from 3 plants were harvested 24 hours after treatment, immediately frozen in liquid nitrogen and then stored at -80°C before further analysis.
Plant fitness parameters
Leaf (5th and 11th) area was measured by using a ruler. Leaf dry weight was measured on a microbalance (0.001 g resolution) after drying the leaves in an oven at 60°C. Flowering time was determined as the time of first flower appearance after treatment. To define seed production, plants were watered every other day until they stopped producing new flowers. Inflorescences were harvested when plants had finished flowering and the seeds were weighed on a microbalance with a 0.0001 g resolution.
RNA extraction and RT-qPCR
Total RNA was isolated as described in Oñate-Sánchez and Vicente-Carbajosa, 2008. DNase treatment was performed by using DNase I (Fermentas) at the concentration of 0.5U/μg RNA. To convert DNA-free total RNA into cDNA, RevertAid H minus Reverse Transcriptase (Fermentas) was used. PCR reactions were made in optical 96-well plates (Applied Biosystems) with ABI PRISM® 7900 HT sequence detection system by using SYBR® Green to detect the synthesis of double-stranded DNA. The following thermal profile was used: 50°C for 2 min, 95°C for 10 min, 40 cycles of 95°C for 15 s and 60°C for 1 min. Amplicon dissociation curves were monitored after cycle 40 by heating from 60 to 95°C with a ramp speed of 1°C/min. Transcript levels were calculated relative to the reference gene PP2AA3 (Czechowski et al., 2005) using the 2-ΔΔCT method previously described (Livak and Schmittgen, 2001). The AGI numbers of the studied genes are At1g67710 (ARR11), At5g44420 (PDF1.2), and At1g13320 (PP2AA3). Fold change was calculated relative to the mock treatment. A one-way and two-way ANOVA was performed on fold changes to determine the statistical significance of differences in expression levels of ARR11 and PDF1.2, respectively
Pathogen bioassay
Botrytis cinerea bioassay has been performed according to Van Wees et al. (2013), with some modification. Botrytis cinerea strain B05.10 was grown for 2 weeks on half-strength potato dextrose agar (PDA; Difco Laboratories, Leeuwarden, the Netherlands) plates containing penicillin (100 µg mL-1) and streptomycin (200 µg mL-1) at room temperature. B. cinerea spores were collected, filtered through glass wool, and re-suspended in half-strength potato dextrose broth (PDB; Difco Laboratories, Leeuwarden, the Netherlands) to a final density of 1x105 spores mL-1. After a 3-h incubation period, 5-week-old plants were inoculated by applying 5µl droplets of the spore suspension to six leaves of each plant. Plants were infected after 24 hrs by hormone/mock treatments, performed as in par. 2.2. Plants were placed in a closed box to increase relative humidity to 100% to stimulate the infection. Three days after B. cinerea inoculation, the symptoms were scored in five disease severity classes ranging from Stage I, lesion 2 mm; stage II, lesion 2 mm + chlorosis; stage III, lesion 2–4 mm + chlorosis; stage IV, lesion > 4 mm + chlorosis; stage V (lesion > 4-5 mm with tissue maceration). Percentage of leaves in each class was calculated per plant (χ2 test; n=24 plants per line).
ROS detection
ROS detection was performed as described in Proietti et al., 2019, on leaves of Col-8 and arr11 plants, three days after B. cinerea infection, with or without hormone treatment Briefly, ROS production was detected by using 2’,7’-dichlorofluorescein diacetate (DCFH2-DA; Sigma Aldrich, St. Louis, MO, USA), that is oxidized to highly fluorescent dichlorofluorescein (DCF) when ROS are present. Two leaves from each of six 5-week-old Col-8 and arr11 plants were collected. One leaf from each plant was incubated at room temperature in 20 mM DCFH2-DA in 10 mM Tris-HCl solution (pH 7.4) for 45 min in the dark. As a negative technical control, the other leaf was incubated in 10 mM Tris-HCl (pH 7.4) only, under the same conditions. After the staining, the samples were washed three times in 10 mM Tris-HCl (pH 7.4) for 10 min to eliminate the excess of fluorophore and finally mounted on glass slides. Fluorescence was then observed under a LSM 710 confocal microscope (Carl Zeiss Microscopy GmbH, Jena, Germany) with Plan Neofluar 20/1.30 objective. Two laser excitations lines were used (i.e., 488 nm for probe detection and 561 nm for chlorophyll auto-fluorescence). Data were processed using Image J software (http://rsbweb.nih.gov/ij/).
Thiobarbituric acid reactive substance measurement
TBARS levels were used to assess lipid peroxidation following the protocol described in Bertini et al., 2019 and in Proietti et al., 2021, on leaves of Col-8 and arr11 plants, three days after B. cinerea infection, with or without hormone treatment. Briefly, four hundred milligrams of frozen leaves were finely ground using a mortar and pestle under continuous addition of liquid nitrogen. The powder was resuspended in 3 mL of trichloroacetic acid (TCA), 0.1%, and mixed on the vortex until homogenized. Following centrifugation at 13,000 rpm for 10 min, 400 µL of the supernatant (or 0.1% TCA for the blank) was added either to 1 mL of 0.5% TBA in 20% TCA (+TBA solution) or to 1 mL of 20% TCA (–TBA solution) (dilution factor 1:3.5). Samples were then incubated at 80°C for 30 min and cooled on ice. After centrifugation at 13500 rpm for 5 min, the absorbance was measured both at 532 nm, that represents the maximum absorbance of the TBA–TBARS complex, and at 600 nm to allow correction of non-specific turbidity. To calculate the TBARS equivalent (nmol mL–1), the ϵµM (0.155 µM−1 cm−1) of malondialdehyde (MDA), one of the main products of membrane damage, was used according to the following formula:
[A/εµM MDA] × dilution factor
where A = [(A532nm (+TBAsol) − A600nm (+TBAsol))] − [(A532nm (−TBAsol) − A600nm (−TBAsol)]
Results
To investigate the impact of ARR11 on plant fitness following SA/JA/CK treatment, leaf area, dry weight, day of flowering and seed production were measured in the arr11 mutant and Col-8, after single and combined treatments with SA, MeJA, CK (Figure 1). A general reduction of fitness parameters, although not always statistically significant, was observed after SA and JA treatments in both Col-8 and arr11, compared to mock. Besides, CK was able to significantly increase leaf area, dry weight, flowering time and seed production in both Col-8 and arr11 mutant, compared to mock. This positive effect was observed also after SA/MeJA/CK treatment, although slightly reduced. However, in general, the data did not show a significant difference between Col-8 and arr11 across all parameters with mock or hormone treatment.
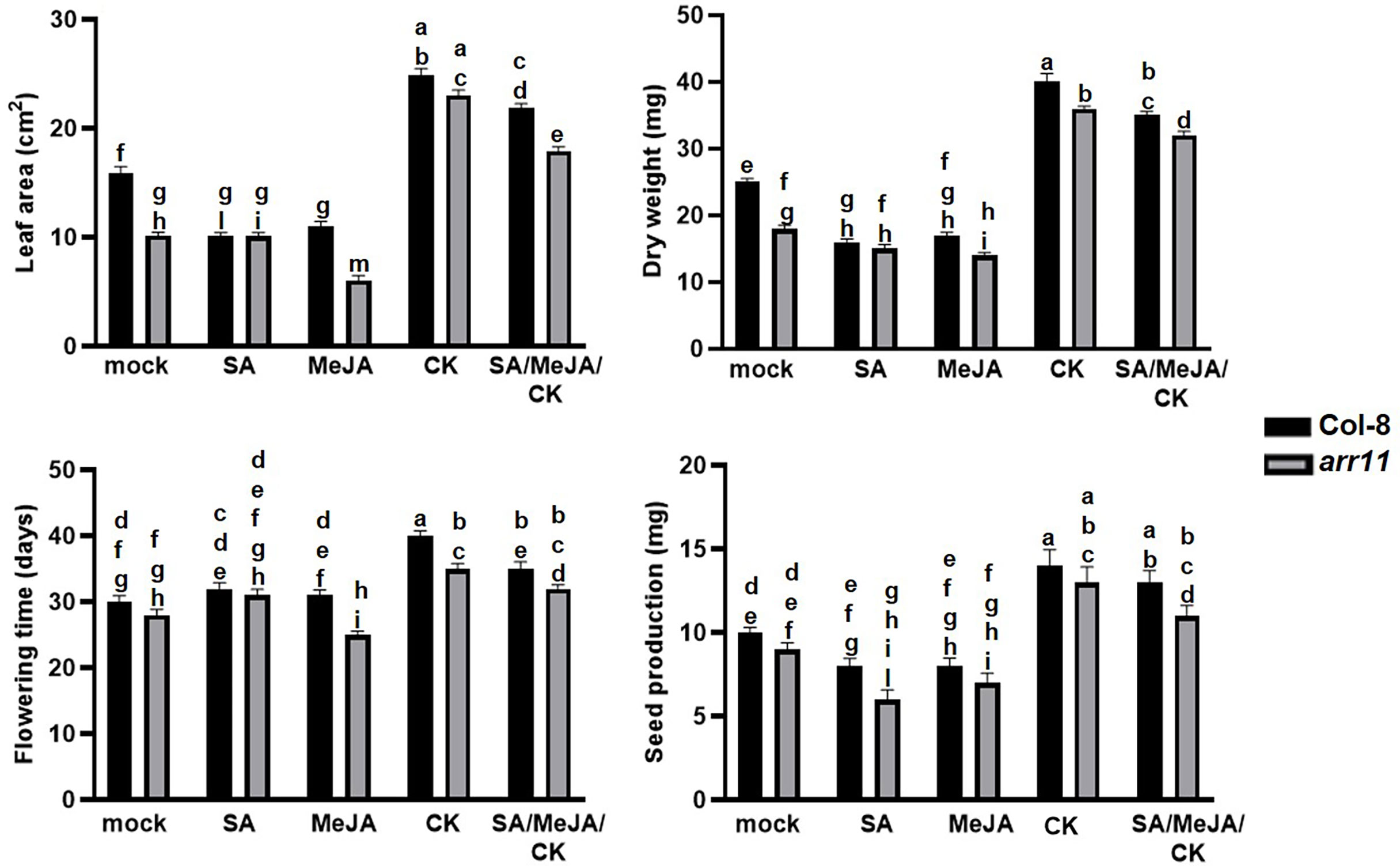
Figure 1 Fitness parameters of Arabidopsis Col-8 and arr11 mutant. Leaf area (cm2), dry weight (mg), flowering time (days), and seed production (mg) were analyzed in Col-8 and arr11 plants after single and triple hormone treatment. Error bars represent SEM. Letters indicates a statistically significant difference between treatments and genotypes (two-way ANOVA; p< 0.0001, n= 15). The experiments have been repeated three times with similar results.
We further investigated the involvement of ARR11 in the plant’s response to hormone treatments at molecular level. Firstly, ARR11 gene expression was analyzed in Col-8 after treatment with SA, MeJA, CK alone as well as in combination. As shown in Figure 2A, while single hormone treatment had no effect on ARR11 expression, SA/MeJA/CK treatment had a significant synergistic effect on ARR11 expression. This result highlights a great impact of the triple hormone treatment on ARR11 expression. Furthermore, since the JA pathway was perturbed in the arr11 mutant (Proietti et al., 2018), we wondered if the SA/MeJA/CK treatment could also impact it. Thus, the expression of the JA marker gene PDF1.2 was investigated (Figure 2B). MeJA was able to increase PDF1.2 expression in both Col-8 and arr11, although more efficiently in the mutant. After CK treatment, the marker significantly increased in arr11 mutant only. The triple hormone treatment had a synergistic effect on PDF1.2 expression in both Col-8 and arr11 mutant, compared to the single hormone treatment. This effect was definitely more pronounced in arr11 compared to Col-8.
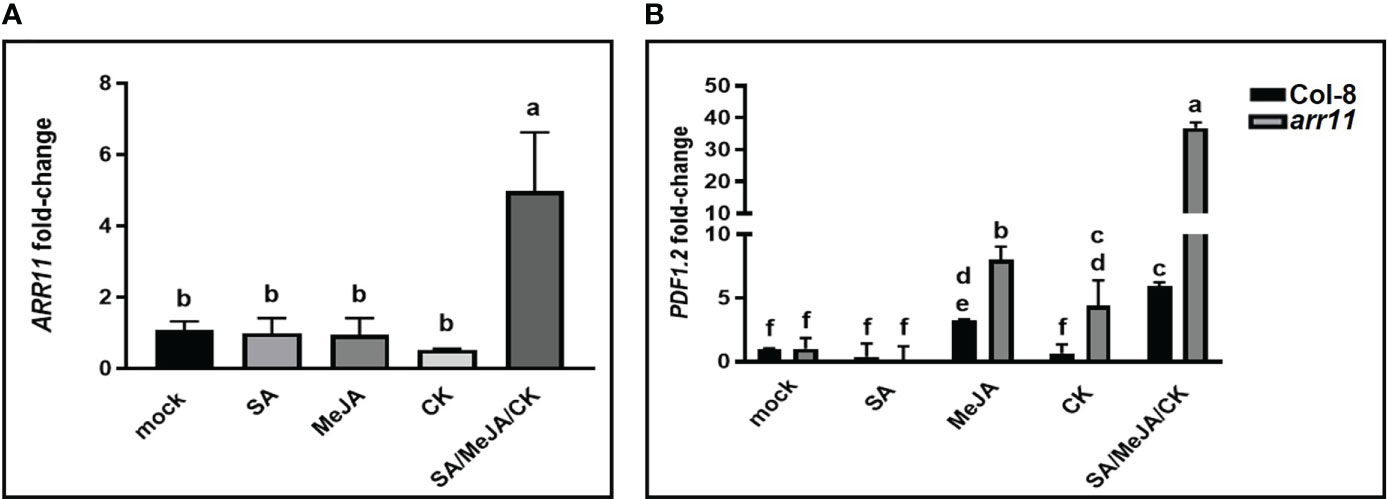
Figure 2 Gene expression analysis after hormone treatments. qRT-PCR analysis of ARR11 (A) in leaves of Col-8 and of PDF1.2 (B) in leaves of Col-8 and arr11, that were treated with a mock solution or with SA, MeJA, CK, SA/MeJA/CK. Fold change is relative to the expression in mock-treated plants and normalized to the reference gene PP2AA3. Gene expression analyses was performed 24 h after hormone treatment of 5-week-old plants. Letters indicates statistically significant differences between treatments and mock. One-way ANOVA, p< 0.05 (A); two-way ANOVA, p< 0.0001 (B).
We then tested the effect of single and combined SA/MeJA/CK treatment on B. cinerea resistance in both Col-8 and arr11 mutant. In the mock, arr11 mutant showed more susceptibility to B. cinerea compared to Col-8 and this result is in agreement with Proietti et al., 2018. The effect of single hormone treatment was visible in both Col-8 and ar11 mutant, although with different magnitude. The most interesting result is that the combination SA/MeJA/CK led to an increased resistance to B. cinerea, compared to single treatments and to mock, in both Col-8 and arr11 mutant (Figure 3). Noteworthy, under triple treatment, this resistance was strongly enhanced in arr11 mutant compared to Col-8. The increased resistance to B. cinerea could be supported by the higher expression of PDF1.2 in this sample (Figure 2B).
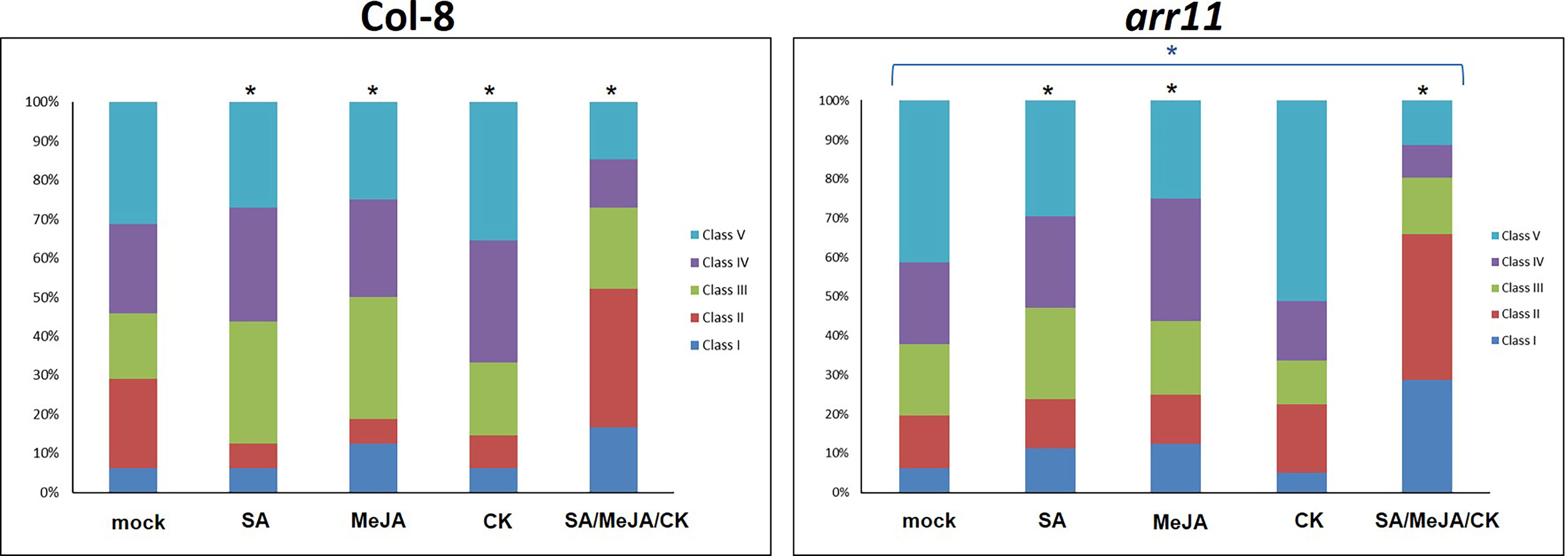
Figure 3 B cinerea bioassay in Col-8 and arr11. Distribution of disease symptoms of leaves of Col-8 and arr11, 3 days after inoculation with B cinerea. The bars indicate the frequency distribution of disease symptoms. Disease rating is expressed in 5 classes: I, from no necrotic lesion visible to lesion smaller than 3 mm; II, lesion between 4 mm to 1 cm; III, lesion bigger than 1 cm; IV, lesion between 1 cm and 1.5 cm; V, necrotic leaf. A black asterisk above the bars indicates significant differences between mock and each treatment (χ2-test, p-value<0.05). The blue asterisk above the bracket indicates significant differences in response to any treatment between arr11 and Col-8.
In order to test if the triple treatment has an effect on reducing B. cinerea-induced oxidative stress, ROS as well as lipid peroxidation were analyzed in both Col-8 and arr11, after single and triple treatment (Figure 4). ROS were detected in five-week-old leaves, after incubation with 2,7-dichlorofluorescein diacetate (2,7-DCFH2-DA), a compound largely used as ROS-sensitive dyes (Proietti et al., 2019). This molecule diffuses through the plasma membrane into the cytoplasm and is deacetylated by intracellular esterase before being oxidized by ROS to produce the green, fluorescent dye 2’,7’-DCF. Originally, it was assumed that oxidation of DCFH2 to DCF was limited to H2O2, but recent data has demonstrated that other ROS such as hydroxyl radical, hydroperoxides, and peroxynitrite may also oxidize DCFH2, albeit with much lower sensitivity than H2O2 (Proietti et al., 2019). The green spots that mark the presence of ROS, seemed very abundant in Col-8 infected by B. cinerea, while they were strongly reduced after MeJA and the triple treatment. On the other hand, in arr11 mutant the effect did not seem to be hormone dependent since ROS were already low in the mock (representative photos are in Figure 4A and Supplementary Figure 1) The effectiveness of SA/MeJA/CK treatment in reducing lipid peroxidation was tested by TBARS assay (Figure 4B). The assay involves the reaction of lipid peroxidation products, primarily malondialdehyde (MDA) with thiobarbituric acid (TBA), which leads to the formation of MDA-TBA2 adducts called TBARS. Triple hormone treatment was able to reduce B. cinerea-induced lipid peroxidation with more pronounced effect in the arr11 mutant than Col-8.
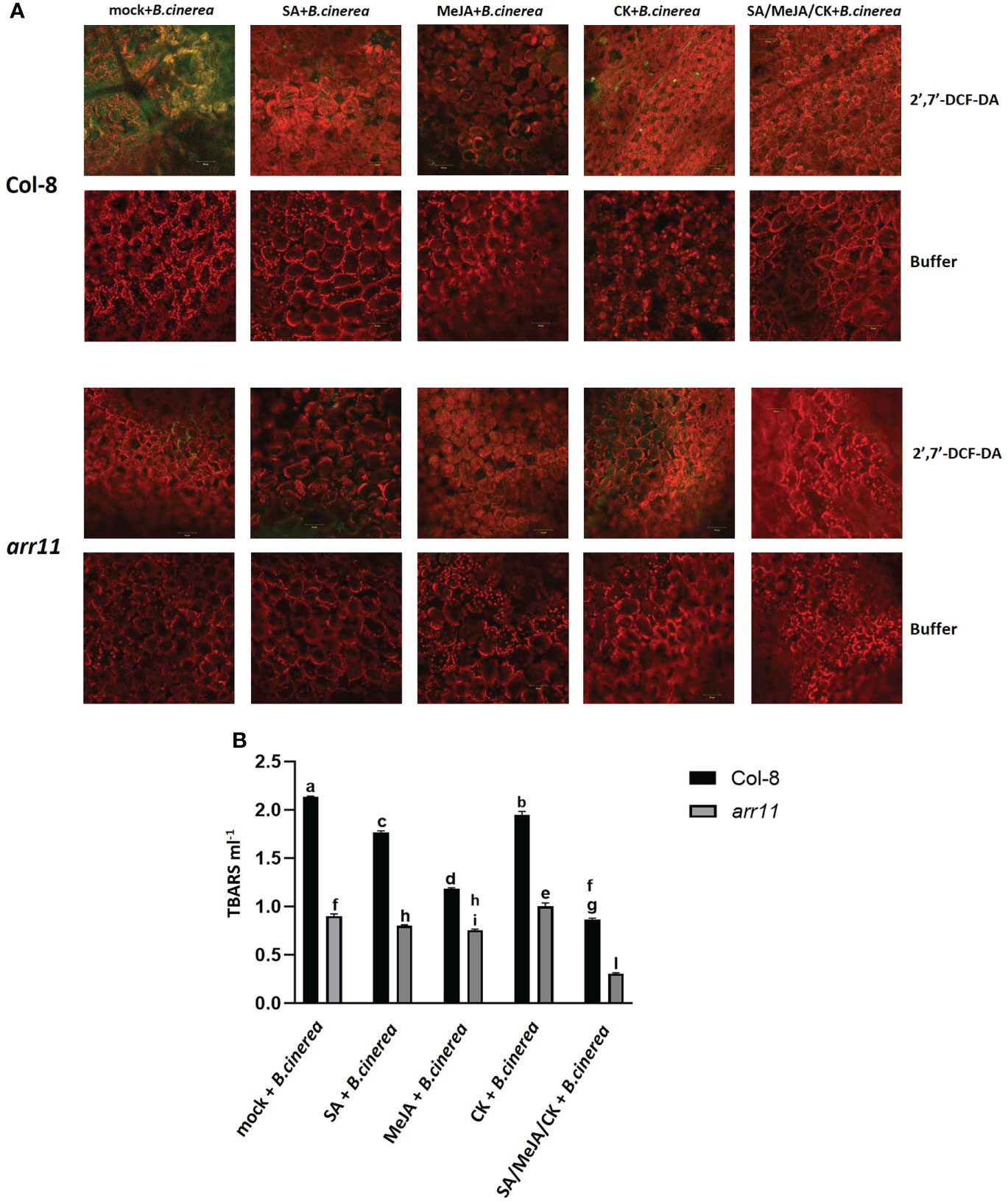
Figure 4 Detection of ROS and lipid peroxidation in Arabidopsis Col-8 and arr11 leaves. (A) Detection of ROS on Col-8 and arr11 leaves was carried out by using 2’,7’-DCFH2-DA or buffer (negative technical control). Fluorescence was observed under an LSM 710 confocal microscope with Plan Neofluar 20/1.30 objective. Laser excitations line was used, i.e., 488 for probe detection (green) and 561 nm for chlorophyll autofluorescence (red). Bar corresponds to 50 µm. The representative merged images are shown. (B) The level of TBARS was used to assess lipid peroxidation measuring the absorbance of MDA–TBA complex at 532 and 600 nm. Letters indicate statistically significant difference between different treatments and genotypes (two-way ANOVA, Tukey’s test p-value< 0.0001). Error bars represent means ± SDs (n = 3).
Discussion
Plant hormones play pivotal role in several aspects of plant life and their signaling pathways can cross-communicate, leading to an optimization of plant adaptive responses. In Arabidopsis, one of the best investigated cross-talk is between SA and JA, with several other hormones playing as modulator of the backbone (Aerts et al., 2020). Among the latter are CKs, which are primarily involved in plant growth and development but also in stress responses (Choi et al., 2010; Robert-Seilaniantz et al., 2011; Giron et al., 2013; O’Brien and Benková, 2013). CK signaling network involves a number of ARR, among which is ARR11, which also recently emerged as a new SA/JA crosstalk regulator (Argyros et al., 2008; Proietti et al., 2018). Here, we investigated the impact of the triple hormonal treatment with SA, JA and CK on plant growth and JA-mediated defense as well as the involvement of ARR11 in both physiological responses. We found that SA/JA/CK treatment is able to restore the negative impact of SA and JA on growth parameters and, most interestingly, in general ARR11 positively impact plant fitness, both under hormone treatment and in mock (Figure 1). This result corroborated previous findings in which the lack of type-B ARR family members was deleterious for plant fitness and development (Imamura et al., 2003). At molecular level, we showed that the triple hormone treatment increased ARR11 transcripts, suggesting a need for boosting under the effect of SA/JA/CK (Figure 2A). Moreover, SA/JA/CK had a great impact on the JA-responsive marker gene PDF1.2, and when ARR11 was defective, this effect was even more pronounced (Figure 2B). Noteworthy, JA and CK alone were already able to increase PDF1.2 expression in arr11 mutant compared to Col-8 (Figure 2B), and the enhanced outcome triggered by the triple treatment could be due by a synergistic effect of CK and JA. As recently reported in rice, CK treatment is able to boost JA signaling pathway (Zhang et al., 2022). Besides the role as negative regulator in SA/JA crosstalk (Proietti et al., 2018), our results suggest that ARR11 could be a negative regulator of the JA signaling pathway under the effect of combined SA/JA/CK treatment. The JA-pathway is well known to be activated against the necrotrophic pathogens, such as B. cinerea (Pieterse et al., 2012). We found that SA/JA/CK increased the resistance against B. cinerea and this effect was even stronger when ARR11 was defective (Figure 3), suggesting that ARR11 could play a role as negative regulator of the resistance against B. cinerea in these conditions. Furthermore, it is well known that under biotic stress plants increases ROS formation (Huang et al., 2019). As reported in Zwack et al., 2016, ARR11 is downregulated in response to oxidative stress, allowing improved stress tolerance. In our work, we observed that SA/JA/CK treatment was able to mitigate ROS generation, especially in Col-8. Lipid peroxidation induced by B. cinerea was also reduced by the triple hormone treatment and this effect was even more pronounced when ARR11 was defective (Figures 4A, B).
In conclusion, taken together our results proved the importance of ARR11 in plant defense related processes. Future research will be performed to undisclosed the mechanism of action of ARR11 in multiple hormone pathways, as well as in response to plant pathogens.
Data availability statement
The raw data supporting the conclusions of this article will be made available by the authors, without undue reservation.
Author contributions
SP and CC designed the study. SP, GF, LB, and EB conducted the experiments. GF and LB analyzed the data. SP and CC wrote the manuscript. All authors contributed to the article and approved the submitted version.
Funding
The authors acknowledge the PRIN grant (PROSPECT 2017JLN833_005) by Ministero dell’Istruzione, dell’Università e della Ricerca.
Conflict of interest
The authors declare that the research was conducted in the absence of any commercial or financial relationships that could be construed as a potential conflict of interest.
Publisher’s note
All claims expressed in this article are solely those of the authors and do not necessarily represent those of their affiliated organizations, or those of the publisher, the editors and the reviewers. Any product that may be evaluated in this article, or claim that may be made by its manufacturer, is not guaranteed or endorsed by the publisher.
Supplementary material
The Supplementary Material for this article can be found online at: https://www.frontiersin.org/articles/10.3389/fpls.2022.995178/full#supplementary-material
Supplementary Figure 1 | Detection and quantification of ROS, as detected in . (A) Detection of ROS on Col-8 and arr11 leaves was carried out by using 2’,7’-DCFH2-DA or buffer (negative technical control). Fluorescence was observed under an LSM 710 confocal microscope with Plan Neofluar 20/1.30 objective. Green fluorescence only is showed. Bar corresponds to 50 µm. (B). Quantification of green fluorescence detected by using 2’,7’-DCFH2-DA in Col-8 and arr11 leaves, based on images of panel A. Heatmap of integrated density that sums all the pixels within the analyzed area giving a total value is shown. Quantification has been performed by Image J, version 1.53s.
References
Aerts, N., Chhillar, H., Ding, P., Van Wees, ,.S. C. M. (2022). Transcriptional regulation of plant innate immunity. Essays Biochem. EBC20210100. doi: 10.1042/ebc20210100
Aerts, N., Pereira Mendes, M., Van Wees, S. C. M. (2020). Multiple levels of crosstalk in hormone networks regulating plant defense. Plant J. 105 (2), 489–504. doi: 10.1111/tpj.15124
Argueso, C. T., Ferreira, F. J., Epple, P., To, J. P. C., Hutchison, C. E., Schaller, G. E., et al. (2012). Two-component elements mediate interactions between cytokinin and salicylic acid in plant immunity. PloS Genet. 8 (1), e1002448. doi: 10.1371/journal.pgen.1002448
Argyros, R. D., Mathews, D. E., Chiang, Y.-H., Palmer, C. M., Thibault, D. M., Etheridge, N., et al. (2008). Type b response regulators of arabidopsis play key roles in cytokinin signaling and plant development. Plant Cell 20 (8), 2102–2116. doi: 10.1105/tpc.108.059584
Bertini, L., Focaracci, F., Proietti, S., Papetti, P., Caruso, C. (2019). Physiological response of Posidonia oceanica to heavy metal pollution along the tyrrhenian coast. Funct. Plant Biol. 46 (10), 933. doi: 10.1071/fp18303
Choi, J., Huh, S. U., Kojima, M., Sakakibara, H., Paek, K.-H., Hwang, I. (2010). The cytokinin-activated transcription factor ARR2 promotes plant immunity via TGA3/NPR1-dependent salicylic acid signaling in arabidopsis. Dev. Cell 19 (2), 284–295. doi: 10.1016/j.devcel.2010.07.011
Czechowski, T., Stitt, M., Altmann, T., Udvardi, M.K., Scheible, W.-R. (2005). Genome-wide identification and testing of superior reference genes for transcript normalization in arabidopsis. Plant Physiol. 139 (1), 5–17. doi: 10.1104/pp.105.063743
Giron, D., Frago, E., Glevarec, G., Pieterse, C. M. J., Dicke, M. (2013). Cytokinins as key regulators in plant–microbe–insect interactions: Connecting plant growth and defence. Funct. Ecol. 27 (3), 599–609. doi: 10.1111/1365-2435.12042
Hickman, R., Mendes, M. P., Van Verk, M. C., Van Dijken, A. J. H., Di Sora, J., Denby, K., et al. (2019). Transcriptional dynamics of the salicylic acid response and its interplay with the jasmonic acid pathway. bioRxiv. 742742. doi: 10.1101/742742
Hosoda, K., Imamura, A., Katoh, E., Hatta, T., Tachiki, M., Yamada, H., et al. (2002). Molecular structure of the GARP family of plant myb-related DNA binding motifs of the arabidopsis response regulators. Plant Cell 14 (9), 2015–2029. doi: 10.1105/tpc.002733
Huang, X., Hou, L., Meng, J., You, H., Li, Z., Gong, Z., et al. (2018). The antagonistic action of abscisic acid and cytokinin signaling mediates drought stress response in arabidopsis. Mol. Plant 11 (7), 970–982. doi: 10.1016/j.molp.2018.05.001
Huang, H., Ullah, F., Zhou, D.-X., Yi, M., Zhao, Y. (2019). Mechanisms of ROS regulation of plant development and stress responses. Front. Plant Sci. 10. doi: 10.3389/fpls.2019.00800
Imamura, A., Hanaki, N., Nakamura, A., Suzuki, T., Taniguchi, M., Kiba, T., et al. (1999). Compilation and characterization of arabiopsis thaliana response regulators implicated in his-asp phosphorelay signal transduction. Plant Cell Physiol. 40 (7), 733–742. doi: 10.1093/oxfordjournals.pcp.a029600
Imamura, A., Kiba, T., Tajima, Y., Yamashino, T., Mizuno, T. (2003). In vivo and in vitro characterization of the ARR11 response regulator implicated in the His-to-Asp phosphorelay signal transduction in arabidopsis thaliana. Plant Cell Physiol. 44 (2), 122–31. doi:10.1093/pcp/pcg014
Jiang, C.-J., Shimono, M., Sugano, S., Kojima, M., Liu, X., Inoue, H., et al. (2013). Cytokinins act synergistically with salicylic acid to activate defense gene expression in rice. Mol. Plant-Microbe Interactions® 26 (3), 287–296. doi: 10.1094/mpmi-06-12-0152-r
Kushwah, S., Jones, A. M., Laxmi, A. (2011). Cytokinin interplay with ethylene, auxin, and glucose signaling controls arabidopsis seedling root directional growth. Plant Physiol. 156 (4), 1851–1866. doi: 10.1104/pp.111.175794
Liang, Y., Wang, X., Hong, S., Li, Y., Zuo, J. (2012). Deletion of the initial 45 residues of ARR18 induces cytokinin response in arabidopsis. J. Genet. Genomics 39 (1), 37–46. doi: 10.1016/j.jgg.2011.12.004
Livak, K.J., Schmittgen, T.D. (2001). Analysis of relative gene expression data using real-time quantitative PCR and the 2−ΔΔCT method. Methods, 25 (4), 402–408. doi:10.1006/meth.2001.1262
O’Brien, J. A., Benková, E. (2013). Cytokinin cross-talking during biotic and abiotic stress responses. Front. Plant Sci. 4. doi: 10.3389/fpls.2013.00451
Oñate-Sánchez, L., Vicente-Carbajosa, J. (2008). DNA-free RNA isolation protocols for Arabidopsis thaliana, including seeds and siliques. BMC Res. Notes 1 (1), 93. doi:10.1186/1756-0500-1-93
Osugi, A., Sakakibara, H. (2015). Q&A: How do plants respond to cytokinins and what is their importance? BMC Biol. 13, 102. doi: 10.1186/s12915-015-0214-5
Pieterse, C. M. J., van der Does, D., Zamioudis, C., Leon-Reyes, A., Van Wees, S. C. M. (2012). Hormonal modulation of plant immunity. Annu. Rev. Cell Dev. Biol. 28 (1), 489–521. doi: 10.1146/annurev-cellbio-092910-154055
Proietti, S., Bertini, L., Falconieri, G. S., Baccelli, I., Timperio, A. M., Caruso, C. (2021). A metabolic profiling analysis revealed a primary metabolism reprogramming in arabidopsis glyI4 loss-of-Function mutant. Plants 10 (11), 2464. doi: 10.3390/plants10112464
Proietti, S., Caarls, L., Coolen, S., Van Pelt, J. A., Van Wees, S. C. M., Pieterse, C. M. J. (2018). Genome-wide association study reveals novel players in defense hormone crosstalk in Arabidopsis. Plant Cell Environ. 41 (10), 2342–2356. doi: 10.1111/pce.13357
Proietti, S., Falconieri, G. S., Bertini, L., Baccelli, I., Paccosi, E., Belardo, A., et al. (2019). GLYI4 plays a role in methylglyoxal detoxification and jasmonate-mediated stress responses in arabidopsis thaliana. Biomolecules 9 (10), 635. doi: 10.3390/biom9100635
Robert-Seilaniantz, A., Grant, M., Jones, J. D. G. (2011). Hormone crosstalk in plant disease and defense: More than just JASMONATE-SALICYLATE antagonism. Annu. Rev. Phytopathol. 49 (1), 317–343. doi: 10.1146/annurev-phyto-073009-114447
Sakai, H., Aoyama, T., Oka, A. (2000). Arabidopsis ARR1 and ARR2 response regulators operate as transcriptional activators. Plant J. 24 (6), 703–711. doi: 10.1046/j.1365-313x.2000.00909.x
Thaler, J. S., Humphrey, P. T., Whiteman, N. K. (2012). Evolution of jasmonate and salicylate signal crosstalk. Trends Plant Sci. 17 (5), 260–270. doi: 10.1016/j.tplants.2012.02.010
Tsai, Y.-C., Weir, N. R., Hill, K., Zhang, W., Kim, H. J., Shiu, S.-H., et al. (2012). Characterization of genes involved in cytokinin signaling and metabolism from rice. Plant Physiol. 158 (4), 1666–1684. doi: 10.1104/pp.111.192765
Van Wees, S. C. M., Van Pelt, J. A., Bakker, P. A. H. M., Pieterse, C. M. J. (2013). Bioassays for assessing jasmonate-dependent defenses triggered by pathogens, herbivorous insects, or beneficial rhizobacteria. Methods Mol. Biol. 1011, 35–49. doi: 10.1007/978-1-62703-414-2_4
Wasternack, C., Song, S. (2017). Jasmonates: biosynthesis, metabolism, and signaling by proteins activating and repressing transciption. J. Exp. Bot. 68 (6), 1303–1321. doi: 10.1093/jxb/erw443
Wu, W., Du, K., Kang, X., Wei, H. (2021). The diverse roles of cytokinins in regulating leaf development. Hortic Res. 8, 118. doi: 10.1038/s41438-021-00558-3
Zhang, Y., Li, X. (2019). Salicylic acid: Biosynthesis, perception, and contributions to plant immunity. Curr. Opin. Plant Biol. 50, 29–36. doi: 10.1016/j.pbi.2019.02.004
Zhang, X., Liu, D., Gao, D., Zhao, W., Du, H., Qiu, Z., et al. (2022). Cytokinin confers brown planthopper resistance by elevating jasmonic acid pathway in rice. Int. J. Mol. Sci. 23 (11), 5946. doi: 10.3390/ijms23115946
Keywords: ARR11, plant hormones, Arabidopsis, plant defense, necrotrophic pathogen
Citation: Falconieri GS, Bertini L, Bizzarri E, Proietti S and Caruso C (2022) Plant defense: ARR11 response regulator as a potential player in Arabidopsis. Front. Plant Sci. 13:995178. doi: 10.3389/fpls.2022.995178
Received: 15 July 2022; Accepted: 06 September 2022;
Published: 21 September 2022.
Edited by:
Giulia Malacarne, Fondazione Edmund Mach, ItalyReviewed by:
Jenn To, Bayer Crop Science, United StatesMario Serrano, Center for Genomic Sciences, National Autonomous University of Mexico, Mexico
Copyright © 2022 Falconieri, Bertini, Bizzarri, Proietti and Caruso. This is an open-access article distributed under the terms of the Creative Commons Attribution License (CC BY). The use, distribution or reproduction in other forums is permitted, provided the original author(s) and the copyright owner(s) are credited and that the original publication in this journal is cited, in accordance with accepted academic practice. No use, distribution or reproduction is permitted which does not comply with these terms.
*Correspondence: Silvia Proietti, s.proietti@unitus.it; Carla Caruso, caruso@unitus.it
†These authors share first authorship
‡These authors share senior authorship