- 1Hubei Hongshan Laboratory, Wuhan, Hubei, China
- 2National Key Laboratory of Crop Genetic Improvement, Huazhong Agricultural University, Wuhan, Hubei, China
The Arabidopsis homeodomain transcription factor SHOOT MERISTEMLESS (STM) is crucial for shoot apical meristem (SAM) function, which cooperates with CLAVATA3 (CLV3)/WUSCHEL (WUS) feedback regulation loops to maintain the homeostasis of stem cells in SAM. STM also interacts with the boundary genes to regulate the tissue boundary formation. However, there are still few studies on the function of STM in Brassica napus, an important oil crop. There are two homologs of STM in B. napus (BnaA09g13310D and BnaC09g13580D). In the present study, CRISPR/Cas9 technology was employed to create the stable site-directed single and double mutants of the BnaSTM genes in B. napus. The absence of SAM could be observed only in the BnaSTM double mutants at the mature embryo of seed, indicating that the redundant roles of BnaA09.STM and BnaC09.STM are vital for regulating SAM development. However, different from Arabidopsis, the SAM gradually recovered on the third day after seed germination in Bnastm double mutants, resulting in delayed true leaves development but normal late vegetative and reproductive growth in B. napus. The Bnastm double mutant displayed a fused cotyledon petiole phenotype at the seedling stage, which was similar but not identical to the Atstm in Arabidopsis. Further, transcriptome analysis showed that targeted mutation of BnaSTM caused significant changes for genes involved in the SAM boundary formation (CUC2, CUC3, LBDs). In addition, Bnastm also caused significant changes of a sets of genes related to organogenesis. Our findings reveal that the BnaSTM plays an important yet distinct role during SAM maintenance as compared to Arabidopsis.
1 Introduction
Rapeseed (Brassica napus L., AACC, 2n = 38) is the third-largest oilseed crop worldwide after soy bean and oil palm, accounting for about 13% of the global total production of vegetable oil (USDA ERS, 2021). Shoot apical meristem (SAM) is a crucial structure for forming the aerial organs of plants. Inside it, the dynamic balance between stem cell division and differentiation is required to maintain plant normal growth and development. The cooperation of SAM development will facilitate systematic design for high yield breeds in agriculture (Xue et al., 2020). For example, in B. napus, the development of SAM can affect the plant architecture to generate multi-inflorescence structure (Lu et al., 2022). In addition, by editing the important genes in SAM, we can obtain multilocular silique, which is also a desired trait for the development of high-yield varieties of Brassica (Yang et al., 2018). Therefore, it is of great significance to study the development of SAM for the breed improvement of rapeseed.
The KNOTTED-LIKE HOMEOBOX (KNOX) transcription factors are important regulators for the formation of SAM, which contribute to plant growth and development in all stages (Scofield and Murray, 2006; Barton, 2010; Roth et al., 2018). There are four Class I KNOX genes, i.e., KNOTTED-LIKE FROM ARABIDOPSIS THALIANA 1 (KNAT1)/BREVIPEDICELLUS (BP), KNAT2, KNAT6 and SHOOT MERISTEMLESS (STM). The expression of KNAT1 is limited to the subcutaneous cells of the stem and pedicel, which affects the intermodal development to regulate the plant height (Venglat et al., 2002). KNAT2 and KNAT6 are involved in regulating inflorescence development (Ragni et al., 2008). KNAT6 has also been confirmed to be involved in the formation of organ boundaries during embryogenesis (Belles-Boix et al., 2006). Several research studies revealed that STM functions during embryonic and postembryonic development in the formation and maintenance of SAM (Hay and Tsiantis, 2010). Cell-to-cell communication in plants includes the selective trafficking of transcription factors and other signals through plasmodesmata. The KNOX I family transcription factors, which use this pathway, are essential for stem cell establishment and/or maintenance. CHAPERONIN CONTAINING TCP1 SUBUNIT 8 (CCT8) is a subunit of the chaperonin complex required for gene transport in the KNOX I family, which maintains stem cell homeostasis by affecting the intercellular transport of STM proteins (Xu et al., 2011).
CLAVATA3 (CLV3)/WUSCHEL (WUS) feedback regulation loop is the key pathway regulating the proliferation and differentiation of stem cells in SAM (Schoof et al., 2000). Previous studies have reported that STM uses a different approach from WUS to inhibit the cell differentiation maintaining the function of SAM in Arabidopsis (Brand et al., 2002; Gallois et al., 2002; Lenhard et al., 2002; Cole and Nolte C Werr, 2006). Recent reports found that STM is expressed in the whole meristematic tissue and forms a heterodimer with WUS through direct interaction. The heterodimers bind at the CLV3 promoter site to promote CLV3 expression, confining the WUS-expressing cell population within the organizing center (Su et al., 2020).
Moreover, the interaction between STM and plant hormones is considered a balancing mechanism outside the CLV3/WUS pathway (Song et al., 2017). STM activates the transcription of the cytokinin (CK) synthetic gene ISOPENTENYL TRANSFEREASE7 (IPT7) to increase the cytokinin level in the central zone cells of the SAM (Yanai et al., 2005), whereas it inhibits the gibberellin (GA) synthetic gene GA 20-oxidase1 (GA20ox1) expressed at the base of SAM (Jasinski et al., 2005). Therefore, creating a “high CK and low GA” microenvironment in SAM, which is of great significance to maintaining the SAM functionality (Belles-Boix et al., 2006). FT INTERACTING PROTEIN 3 (FTIP3) and FTIP4 can promote the balance between the maintenance and differentiation of SAM by coordinating intracellular and intercellular transport of STM (Liu et al., 2018). There are also research findings showing that STM inhibits cellular differentiation and endoreduplication, acting through CK and the CK-inducible CYCLIN-D3 (CYCD3) cell cycle regulators, establishing a mechanistic link to cell cycle control which provides sustained mitotic activity to maintain a pool of undifferentiated cells in the SAM (Scofield et al., 2013). STM is also associated with auxin and lateral organ activation-related genes such as AXIAL REGULATOR YABBY 3 (YAB3), ASYMMETRIC LEAVES 1 (AS1), ASYMMETRIC LEAVES 2 (AS2), and JAGGED LATERAL ORGAN (JLO) (Kumaran et al., 2002; Guo et al., 2008; Bureau et al., 2010; Rast MI Simon, 2012).
In addition to regulating meristem identity, STM also functions in boundary specification and leaf shape. In Arabidopsis, the organ-boundary-associated genes CUP-SHAPED COTYLEDON1 (CUC1), CUC2, and CUC3 are required to activate STM expression and the subsequent formation of the SAM during embryogenesis (Vroemen et al., 2003). During embryonic development, CUCs and STM regulate the expression of each other. CUC1 and CUC2 trigger STM expression at the globular stage (Takada et al., 2001). During postembryonic development, STM promotes the expression of CUC1/2/3 (Kwon et al., 2006; Boscá et al., 2011; Spinelli et al., 2011). Research has shown a direct positive transcriptional feedback loop between STM and CUC1, despite their distinct expression patterns in the meristem and organ boundary. Moreover, STM can activate the expression of the CUC1-targeting microRNA miR164c (Scofield et al., 2018). Moreover, in Arabidopsis, it has been reported that the complexity of the leaf shape is related to the expression of STM in the leaves (Piazza et al., 2010). Recent findings revealed that REDUCED COMPLEXITY (RCO) and STM were able to form a complex leaf shape similar to Cardamine hirsuta (Kierzkowski et al., 2019).
The association of SAM with yield traits in tomato, maize, rice, and rapeseed has been confirmed (Doebley, 2004; Elhiti et al., 2013; Ohmori et al., 2013; Fan et al., 2014; Perales et al., 2016). However, few reports are available on STM-related research in B. napus except that altered expression of BnSTM affects the morphology, behavior, and quality of microspore-derived embryos (MDEs) (Elhiti et al., 2013). The present study employed the CRISPR/Cas9 system to obtain the efficient knockout of STM homologs in B. napus through stable Agrobacterium-mediated transformation. Abnormal shoot apex development and cotyledon petiole fusion at the seedling stage was observed in the double mutants. Furthermore, the transcriptomics analysis of the Bnastm mutant was also used to investigate the molecular mechanism.
2 Materials and methods
2.1 Plant materials
The semi‐winter B. napus pure line J9707 was used as the transformation receptor in this study, and the seeds were obtained from the National Engineering Research Center of Rapeseed, Wuhan, China.
2.2 Cultivation conditions
T0 and T1 transgenic plants and WT plants grew in greenhouse (16/8 h of light/dark at 22°C). In the winter-type oilseed rape growing season, the homozygous mutant T3 lines without T-DNA were selected to grow in the experimental farm of Huazhong Agriculture University, Wuhan, China. The field experiment was conducted with a randomized complete block design and repeated three times. 11-12 plants are planted in each row, and the spacing between plants in each row is 21 cm, and the spacing between rows is 30 cm. The field management was performed in line with standard breeding practices.
2.3 Construction of the CRISPR/Cas9 vector and plant transformation
The CRISPR/Cas9 genome-editing system was utilized for gene editing of BnaSTM in this study. To construct the Cas9/sgRNA-expressing binary vectors, sequence-specific sgRNAs in the target gene were selected using the Web-based tool CRISPR-P2.0 (http://crispr.hzau.edu.cn/cgi-bin/CRISPR2). The binary pYLCRIPSR/Cas9 multiplex genome targeting vector system was provided by Prof. Yaoguang Liu (South China Agriculture University, Guangzhou, China) and used for construct assembly according to the method described by Ma et al. (2015). The oligos used in constructing the sgRNA vectors are listed in Table S1. The resulting construct contained a Cas9p expression cassette, sgRNA expression cassettes with target sequences and a hygromycin resistance cassette.
The Agrobacterium tumefaciens-mediated hypocotyl method (Zhou and Fowke 2002) was used to transform the resulting constructs into B. napus (Zhou et al., 2002). The transgenic plants were screened and confirmed by antibiotic selection and PCR.
2.4 Identification of transgenic plants and potential off-targets
The presence of the T-DNA in the construct was identified using the specific primer pairs PB-L/PB-R (Table S1) employing PCR.
The edit detection in transgenic plants using the high-throughput tracking of mutations (Hi-TOM) platform (Yang et al., 2017). The corresponding online tool (http://www.hi-tom.net/hi-tom/) was utilized to analyze the obtained sequencing data and track the mutation in the target sites. The target-specific primer sets are listed in Table S1. Furthermore, the putative off-target sites (7 annotated genes for sgRNA-1 and 10 for sgRNA-2) were identified using CRISPR-P2.0 (http://crispr.hzau.edu.cn/CRISPR2/) against the reference (Brassica napus v4.1).
2.5 Sequence analysis
Multiple sequence alignment of nucleotide and amino acid sequences was performed by ClustalW (http://www.clustal.org/). Motif and domain analysis of amino acid sequences were performed using MEME (https://meme-suite.org/meme/tools/meme), CDD (https://www.ncbi.nlm.nih.gov/Structure/bwrpsb/bwrpsb.cgi) and Pfam (http://pfam.xfam.org/). The homologous amino acid sequences of STM in different species were collected, and the phylogenetic analyses were constructed by MEGA11 software.
2.6 RNA extraction and qRT-PCR
The samples were collected from double mutants (STM-11-7-8-14, STM-14-1-11-9, STM-8-5-1-9), BnaA09.STM single mutants (STM-8-5-10-21, STM-14-8-12-5), BnaC09.STM single mutants (STM-7-7-9-2, STM-11-10-3-6) and WT (J9707). At least three independent extractions were conducted per sample. The sampling period is the 7th day after germination, The sampled tissue is the fused cotyledon petiole containing the SAM for RNA-seq sequencing. We extracted root (seedling stages), leaf (seedling stages), lower stem (bolting stages), bud (bolting stages), flower (flowering stages), SAM (seedling stages), and 7 DAF seeds from WT planted at the same time with the mutant of T3 generation as samples to detect gene expression in different tissues. Total RNA was extracted using the EasyPure Plant RNA Kit (TransGen Biotech, Beijing, China), and cDNA was synthesized using the Transcript RT Kit (TransGen Biotech). To perform the qPCR, the TransStart Top Green qPCR SuperMix Kit (TransGen Biotech) was used employing a CFX384 Real-Time System (Bio-Rad). The relative quantification was performed using the comparative cycle threshold method. The relative amount of PCR product which was amplified using the designed primer sets (Table S1), was normalized to the reference genes, BnaACT7 and BnaUBC9. The data from three biological replicates were analyzed following the relative quantification method (2−ΔΔCT).
2.7 RNA-seq transcriptomic analysis
Samples collection of double mutants and WT, and RNA extraction as described in the above section. The process of cDNA library construction, sequencing, quality control, data filtering, and read mapping to the reference genome (Brassica napus v4.1), identification of differentially expressed genes (DEGs) using DESeq2 and GO. KEGG pathway enrichment analysis of DEGs was performed as described by Shahid et al. (2019). The fragments per kilobase of transcript per million mapped reads (FPKM) were calculated as a measure of gene expression level. The genes with a false discovery rate (FDR) ≤0.05 and an absolute value of log2(fold change) ≥ 1.5 between mutant and wild type (WT) were defined as DEGs. The raw sequence data were submitted in the NCBI Sequence Read Archive (PRJNA769039).
2.8 Paraffin section preparation
Seeds at different germination stages were collected from the double mutants (STM-11-7-8-14, STM-14-1-11-9, STM-8-5-1-9) and WT. Furthermore, immediately fixed for 24 h at 4°C in a fixation solution containing 5% acetic acid, 3% formaldehyde, and 50% ethanol. Embedding, sectioning, and staining with Calcium polystyrene sulphonate were performed as described by Chaplin et al (Chaplin, 1999). Images were obtained using a Nikon ECLIPSE 80i compound microscope.
2.9 Determination of protein and fatty acid content in seeds
The seeds collected from the WT, BnaA09.STM single mutants (aaCC) and double mutants (aacc) lines were used for fatty acid composition, protein and glucosinolate content analysis by near-infrared spectroscopy at the National Engineering Research Center of Rapeseed (Huazhong Agricultural University, Wuhan, China) and the near-infrared spectroscopy analysis model of each substance was constructed by the National Engineering Research Center of Rapeseed.
2.10 Statistical analysis
Statistical analyses were performed using the IBM SPSS Statistics program (version 25). Comparisons of distributions between 2 groups or between 3 groups were made by LSD (Least—Significant Difference) test or Student’s t test. Correlations between 2 variables were tested with Spearman’s rho correlation coefficients.
3 Results
3.1 Molecular cloning and characterization of STM homologs in B napus
Previous studies have shown that STM is a key regulator during SAM development in many plant species. A BLASTP search identified two close homologs of STM in the rapeseed genome (BnaA09g13310D and BnaC09g13580D) and these STM homologs were named BnaA09.STM and BnaC09.STM. DNA and cDNA sequences of STM homologs were cloned to confirm their gene structure as well as check the putative mutations in transformation receptor J9707 (Figure S1). The BnaA09.STM shared 94.87% similarity with BnaC09.STM at the amino acid level.
AtSTM, BnaA09.STM and BnaC09.STM contains four conserved domains in their amino acid sequences, namely, KNOX1, KNOX2, ELK, and HD, indicating that these genes encode proteins with similar functions (Figure S2). The motif analysis of KNOX I genes in different crops indicated that the homologous genes of STM in Brassica species had an additional motif at the front of protein sequence than Arabidopsis, which function was still unknown. This suggests the functional differentiation of STM during the evolution process in Brassica (Figure S3). The phylogenetic analyses revealed that all STM homologs from different Brassica species were clustered together with AtSTM, whereas the STM homologs from other species were clustered on separate branches. Thus, it indicated that STM may have differentiation among different species (Figure S4). The phylogenetic analysis confirmed that BnaA09.STM and BnaC09.STM clustered with BraA09.STM and BolC09.STM and implied that these two BnaSTM homologs might have conserved yet redundant functions, originating from two diploid progenitors.
3.2 Expression analysis of the BnaSTM
To understand the expression pattern of BnaSTM, different tissues, including roots, stems, leaves, flowers, SAM, buds, and 7 days after pollination (contain embryos at the globular stage) seeds, were collected from J9707 for qRT-PCR analysis. It showed that the BnaSTM was highly expressed in SAM and stem (Figure 1A). Although the expression levels of BnaA09.STM and BnaC09.STM has the same trend in different tissues, the expression level of BnaC09.STM was significantly higher than BnaA09.STM.
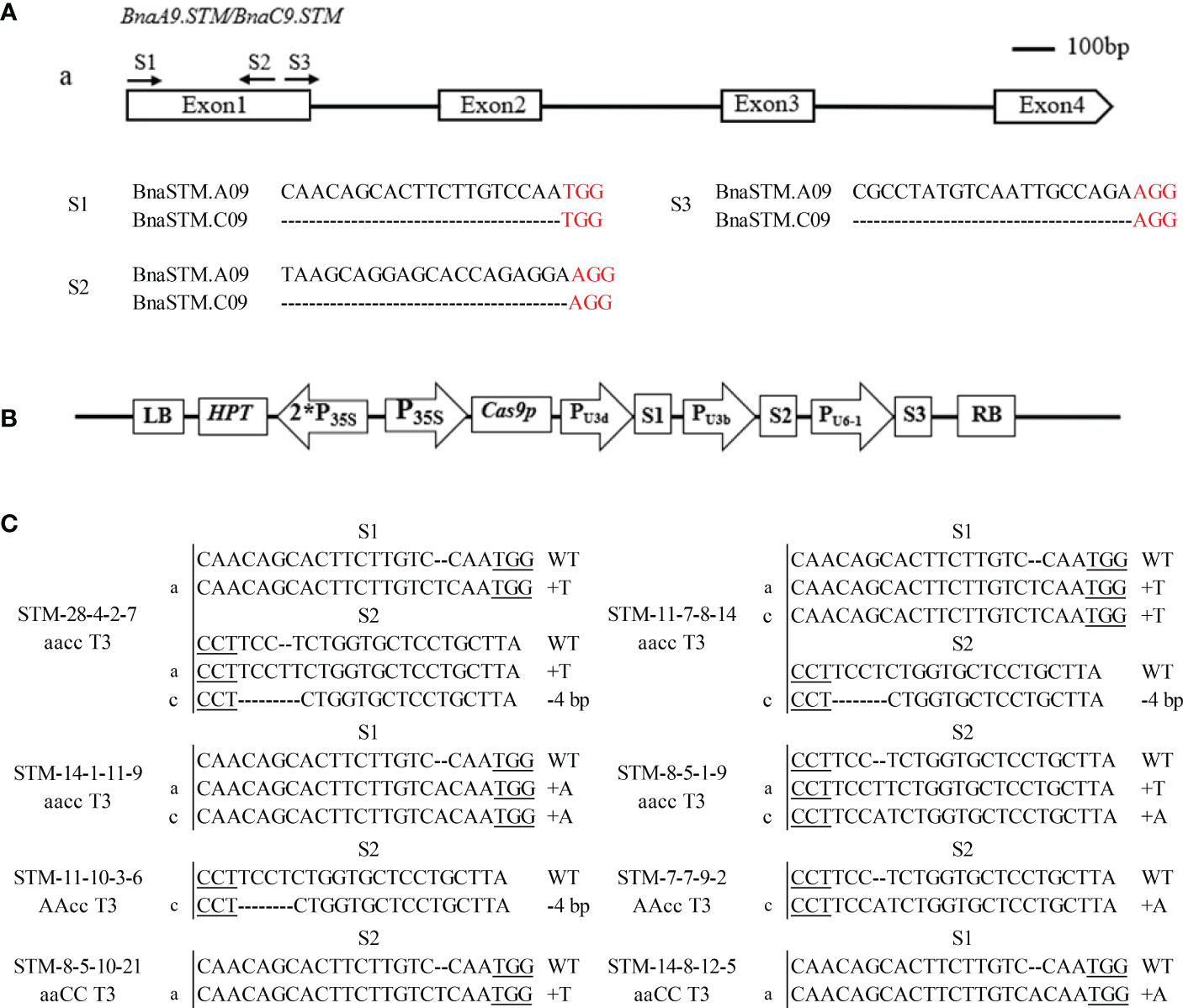
Figure 1 Expression pattern of BnaSTM in rapeseed. (A) qRT-PCR for BnaSTM transcripts in different developmental tissues and stages of J9707. The expression was compared to that of the control BnACT7 gene. SAM, shoot apical meristem. (B) Fragments Per Kilobase of exon model per Million mapped fragments (FPKM) values for BnaSTM in different developmental tissues of ZS11. The data are presented as means ± SE (n ≥ 3); LSD was used to compare the expression of two copies of BnaSTM (**P > 0.01).
Using the data of a recent public RNA-seq data (http://yanglab.hzau.edu.cn/BnTIR) in ZS11 (a reference genome of rapeseed), expression profile of BnaSTM in ZS11 is showed in Figure 1B. Among different tissues, BnaA09.STM and BnaC09.STM has the highest expression levels in stem and bud. Moreover, the expression level of BnaC09.STM was significantly higher than that of BnaA09.STM, which was consistent with the differential expression of the two homologous copies of BnaSTM in J9707.
3.3 Creation of CRISPR/Cas9-targeted mutations in BnaSTM
To generate the Cas9-induced knockout mutations in both copies of BnaSTM, three sgRNAs, viz. sgRNA1 (S1), sgRNA2 (S2), and sgRNA3 (S3), were designed using the CRISPR-P program (Lei et al., 2014). All of these sgRNAs were designed to target the first exon of BnaSTM and ensure the success rate of gene disruptions altering the reading frame. The sgRNAs precisely matched all the BnaSTM copies (Figure 2A). Based on the CRISPR/Cas9 multiple genome editing vectors as previously described by Yang et al. (2018), CRISPR/Cas9 constructs containing these three sgRNAs were generated, in which Cas9 was driven by the 35S promoter (Yang et al., 2018) (Figure 2B).
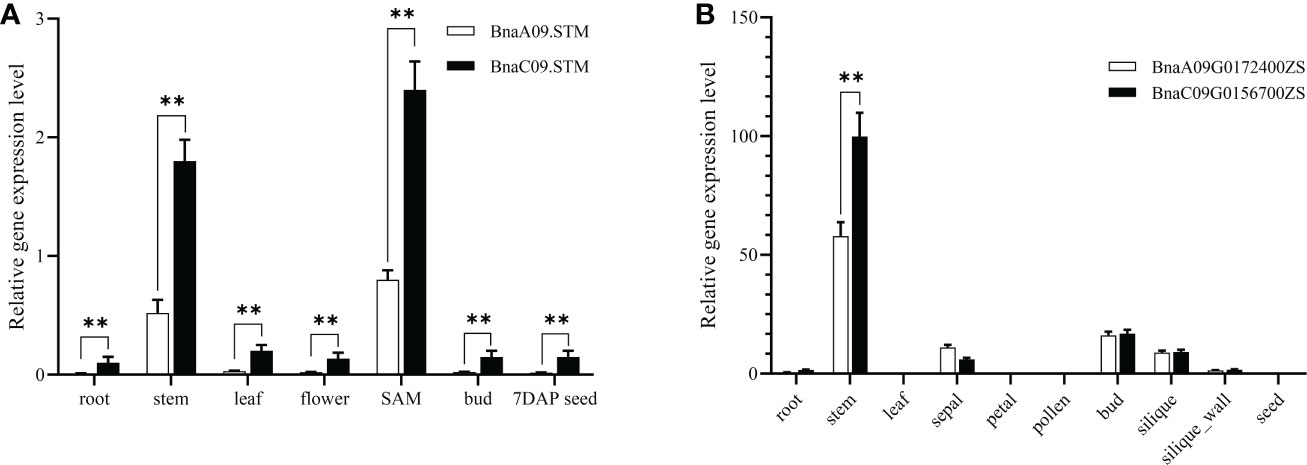
Figure 2 CRISPR/Cas9-induced mutants of BnaSTM in B. napus. (A) The BnaSTM gene model includes four exons (box) separated by three introns (represented by the solid line). The vertical line in the gene model indicates the target site, and the arrow indicates the sgRNA direction. The target sequences are shown with the protospacer adjacent motif (PAM) underlined. (B) The CRISPR/Cas9 construct comprised of a hygromycin resistance cassette (consisting of the hygromycin phosphotransferase coding sequence driven by the cauliflower mosaic virus 35S promoter); a Cas9 expression cassette (comprising the sequence encoding Cas9 driven by P35S); three sgRNAs (S1-S3) driven by the U3d, U3b and U6-1 promoter from Arabidopsis. (C) Sequences at the sgRNA target sites of BnaSTM homozygous mutants in the T3 generation. The PAM is underlined, and nucleotide indels are marked in red, with details labeled at right, ‘a’ and ‘c’ represent the mutated alleles of the target gene on BnaA09.STM and BnaC09.STM, respectively. ‘aaCC’, ‘AAcc’ and ‘aacc’ represent homozygous mutations of the target gene in BnaA09.STM, BnaC09.STM and both copies, respectively.
The resulting CRISPR/Cas9 construct was transformed into J9707 strain using Agrobacterium-mediated transformation. The targeted mutations in all T0-positive transgenic plants were identified using Sanger DNA sequencing of PCR products. Five lines with different targeted mutation types were identified in T0-positive transgenic plants (Table 1).
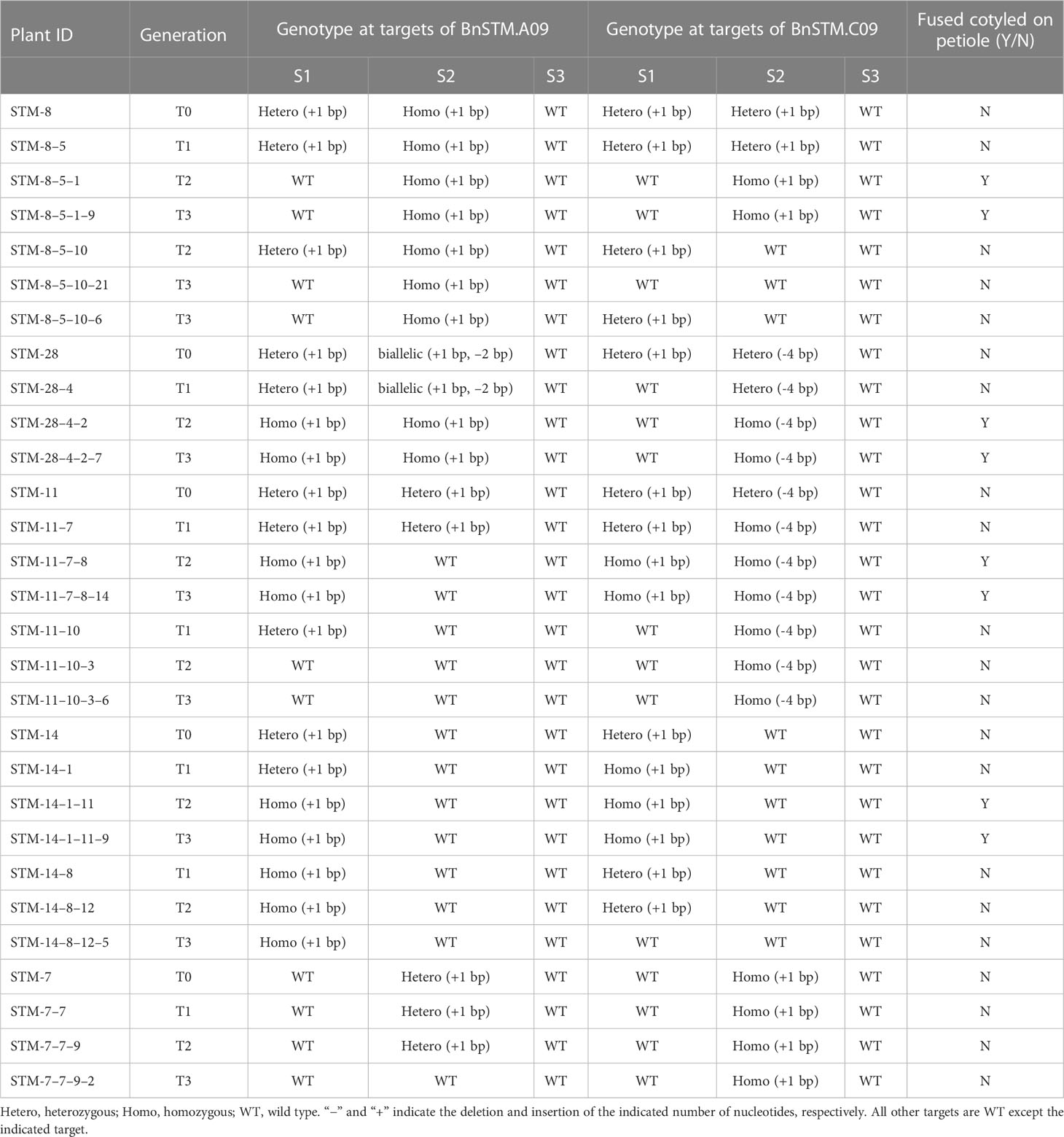
Table 1 Genotypic analysis of BnaSTM mutants and their transmission from T0 to T1, T2, and T3 generations.
To obtain stable lines with targeted mutations, these T0 editing lines of BnaSTM were self-pollinated, producing T1, T2 and T3 progeny. The targeted mutations of progeny from T0 lines were verified by Hi-TOM sequencing analysis of the target sites. The results showed that allelic mutations in the T0 editing lines were transmitted to the following generations, indicating the stable germ-line transmission of Cas9-induced mutations in rapeseed (Table 1). A variable number and type of homozygous mutants with different allelic combinations of these BnaSTM copies were detected, including 7 BnaA09.STM single mutants, 8 BnaC09.STM single mutants and 8 Bnastm double mutants (Figure 2C; Table 1). All of the detected homozygous mutations at the target sites within BnaSTM were predicted, causing frameshifts and resulting in nonfunctional proteins (Figure S5).
3.4 Targeted mutations in BnSTM lead to fusion of the cotyledon petiole in seedling
To dissect the functions of the BnaSTM, some homozygous mutant T2 and T3 lines with different frameshift mutations (Figure S5; Table 1) were chosen for subsequent phenotypic characterization. As expected, all the double mutants could produce a visible knockout phenotype (Figure 3), i.e., a fused cotyledon petiole (the base of the two petioles fused; Figure 3J) or cup-shaped cotyledon (two petioles were fully fused, and the two cotyledons were also fused together; Figure 3M) at 7 days after germination (DAG), while the single mutants showed a comparable phenotype to that of the WT (Figures 3D, G). The longitudinal section of the cotyledon fusion site clearly showed that true leaves were wrapped inside of the Bnastm double mutants (Figure 3M). Eventually, the true leaves of Bnastm double mutants broke out from the cotyledon fusion site about 14 DAG (Figure 3K).
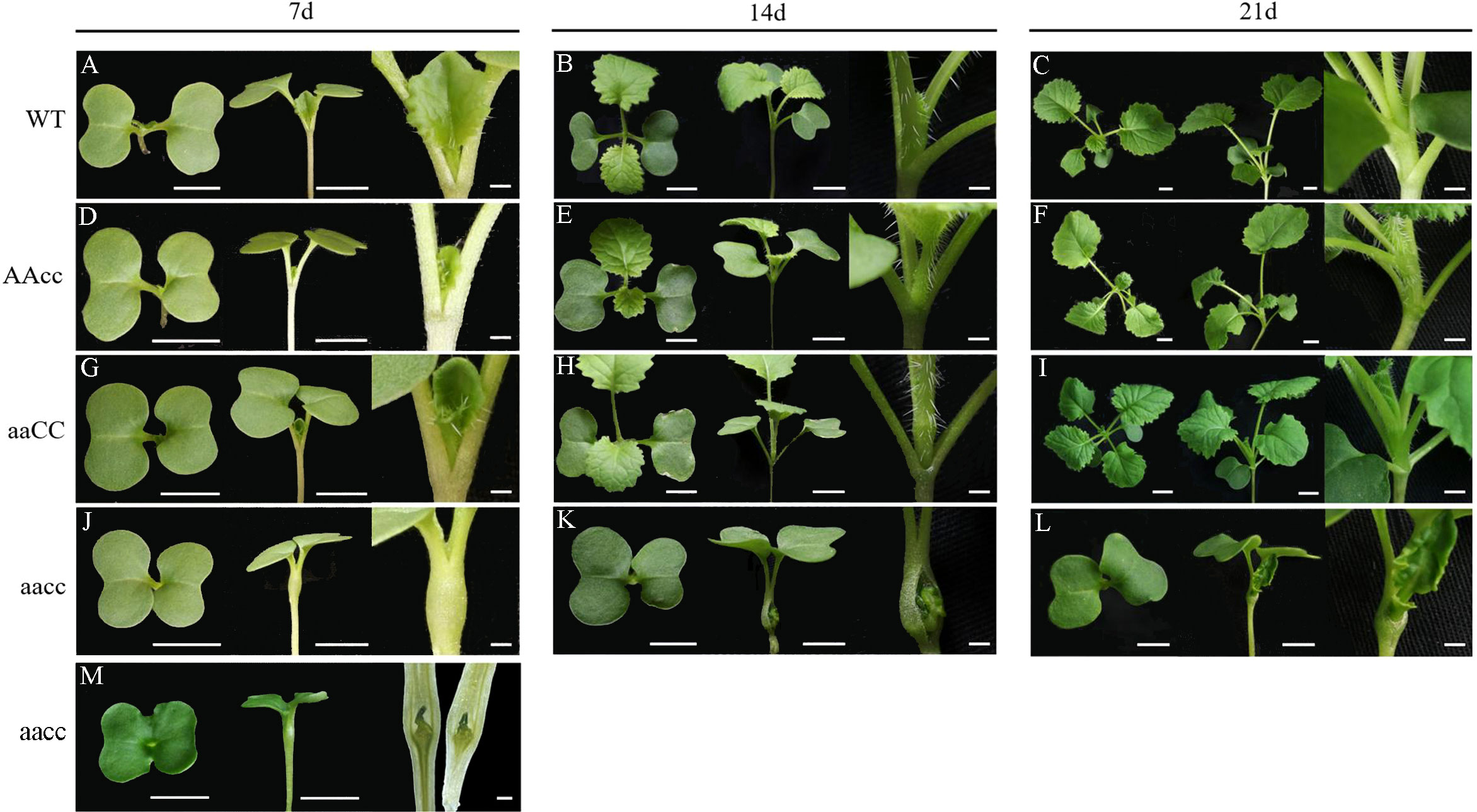
Figure 3 Phenotype of BnaSTM mutant in the T2 generation. (A–C) Top view and side view of WT seedlings at 7, 14, and 21 DAG, respectively; (D–F) Top view and side view of AAcc seedlings at 7, 14, and 21 DAG, respectively; (G–I) Top view and side view of aaCC seedlings at 7, 14, and 21 DAG, respectively; (J–L) Top view and side view of aacc seedlings at 7, 14, and 21 DAG, (M) Part of the aacc material has an extreme cup-shaped cotyledon phenotype. ‘aaCC’, ‘AAcc’ and ‘aacc’ represent homozygous mutations of the target gene in BnaA09.STM, BnaC09.STM and both copies, respectively. Top view and side view scale bars = 1 cm, the Partial view scale bars = 0.01 cm. Section scale bars = 0.5 mm.
From 7 to 21 DAG, the development of mutant seedlings was significantly delayed than that of WT, which may ascribe the fusion of cotyledon petioles physically prevented the outgrowth of the new leaf. However, there was no significant difference between the mutants and WT in the late vegetative and reproductive growth period (Figure 4). Based on these results, we propose that BnaSTM function mainly in early seedling development and BnaA09.STM and BnaC09.STM has a redundant function, and the normal expression of BnaSTM in B. napus is of great significance for the normal separation of cotyledons.
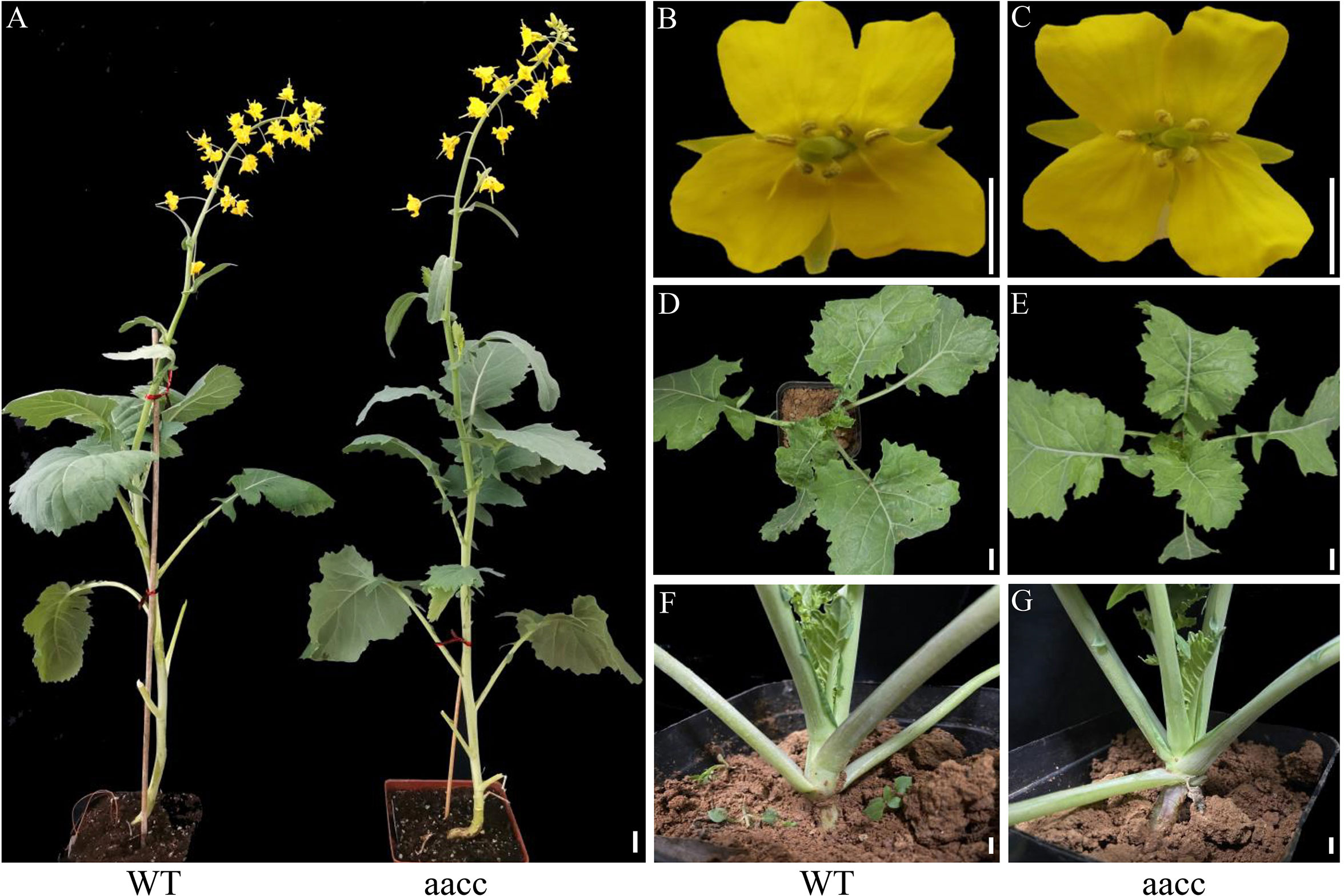
Figure 4 Phenotype of BnaSTM mutant in the T3 generation. (A) Representative plants at the bolting stage of WT and aacc. ‘aacc’ represent homozygous mutations of the target gene in BnaSTM both copies. (B, C) flowers of WT and aacc. (D, E) 40-day old plants of WT and aacc. (F, G) 40-day old plants of WT and aacc. The view Scale bars = 1 cm.
3.5 Cytological observation of SAM in early stage
In Arabidopsis, the stm mutants have abnormal SAM throughout the growth period (Scofield and Murray, 2006). However, in allotetraploid B. napus, the abnormality of SAM in Bnastm double mutant only occurred during early germination process (Figure 5). The Bnastm mutant had no SAM in mature embryo in comparison with WT (Figures 5A, B, H, I). On the third day of germination, a small number of cells appeared at the top of the stem to form a small SAM (Figure 5D). On the fourth day of germination, the development of SAM in the Bnastm double mutant was almost normal, whereas its size was slightly smaller than that of WT (Figure 5E).
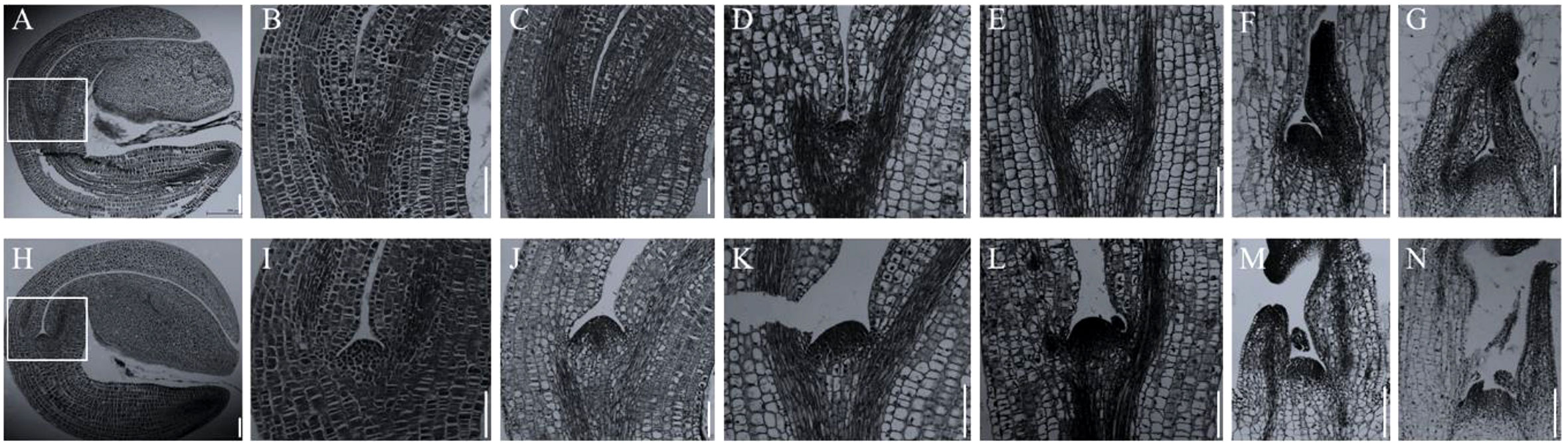
Figure 5 Cytological observation of early stem apical development. (A–G) SAM development of Bnastm double mutant seeds on days 1, 2, 3, 4, 6, and 8 after germination; (H–N) WT observation on SAM development of mutant seeds on days 1, 2, 3, 4, 6, and 8 after germination; (B, I) In (A, H), respectively, the SAM is magnified by 2.5 times; (C, J) Second day after germination; (D, K) Third day after germination; (E, L) Fourth day after germination; (F, M) Sixth day after germination; (G, N) Eighth day after germination. Bar = 164 µm.
Additionally, in comparison with WT, the true leaves formation in Bnastm double mutant were delayed. On the sixth day of germination, the Bnastm double mutant had only one true leaf, while the WT had two visible true leaves (Figures 5F, M). On the eighth day of germination, the true leaves of the Bnastm double mutant were tightly packed inside (Figure 5G). However, the true leaves in WT were not packed together, and there was a large gap between two separated cotyledon petioles (Figure 5N). These results were differed from the phenotype of stm mutant in A. thaliana, indicating that there were more complex mechanisms in allotetraploid B. napus, for the regulation of SAM.
3.6 Off-target activity of CRISPR/Cas9 in T0 and T1 transgenic B. napus plants
To determine whether the fusion of the cotyledon petiole phenotype was resulted from mutations of off-target sites in the edited lines, off-target effects were explored in the edited plants by the CRISPR-P program (Lei et al., 2014). These potential off-target sites are listed in Table S2. S1 and S2 have 7 and 10 putative off-target sites (Table S2), respectively, and all predicted off-target genes and target sites had four base differences, making it difficult to edit effectively, and all predicted off-target genes do not belong to the KNOX gene family. These findings demonstrated that the sgRNAs have high specificity for targeting the BnaSTM in B. napus.
3.7 BnaSTM regulates the expression of genes involved in SAM boundary formation and organogenesis
Given the central importance of STM in SAM and cotyledon petiole development, we performed RNA sequencing on Bnastm double mutants (aacc) and the corresponding WT using fused cotyledon petiole containing the SAM of the 7-days-old-seedlings. A total of 69,134 genes were expressed in seedlings during the same period and were included in the subsequent analysis. Pearson’s correlation coefficient between any two of the three biological replicates was very high (R = 0.93–0.98) in both mutant and WT, which indicated that the transcriptome sequencing data used in this study were highly reliable (Figure S6; Table S3, S4).
Because the expression of many genes in SAM is not high and the expression change is not obvious, so | log2FC | > 1.5 is selected as the threshold of DEGs to expand the number of DEGs and find more genes that may be related to STM. Comparison of transcript abundances fused cotyledon petiole at 7 DAG uncovered 2,084 DEGs between each double mutant and its corresponding WT, which contained 1137 up-regulated genes as well as 947 down-regulated genes. The GO enrichment analysis showed that the two class of the positive regulation of organ growth and the polar auxin transport, which are important for meristem development, were significantly enriched in the up-regulated DEGs. The stem cell fate determination and the commitment were enriched in down-regulated DEGs (Figure S7, S8). And KEGG enrichment analysis of these identified DEGs showed that the down-regulated DEGs in the double mutants were significantly enriched in plant signal transduction pathway (Figure S9).
Among the DEGs, in the case of KNOX genes, except for STM, only the expression levels of one copy of BnaKNAT1 were significantly down-regulated (Figure 6). In addition, the expression level of BnaCCT8, which controls the transport of STM between cells in the meristem, was down-regulated (Figure 6). For the genes related to lateral organ activation, such as BnaYAB3, BnaAS1, BnaAS2 and BnaJLO, only the expression level of BnaAS1 was down-regulated. Previous studies showed that CLV3/WUS maintains stem cell stability through feedback regulation in SAM (Schoof et al., 2000), however, the expression of BnaCLV3 and BnaWUS as key genes in the pathway were not detected in WT and double mutant. Moreover, the BnaCLV1, BnaCLV2 and BnaCRN were the receptor gene of BnaCLV3 signal peptide, and their expression remained unchanged in the double mutant.
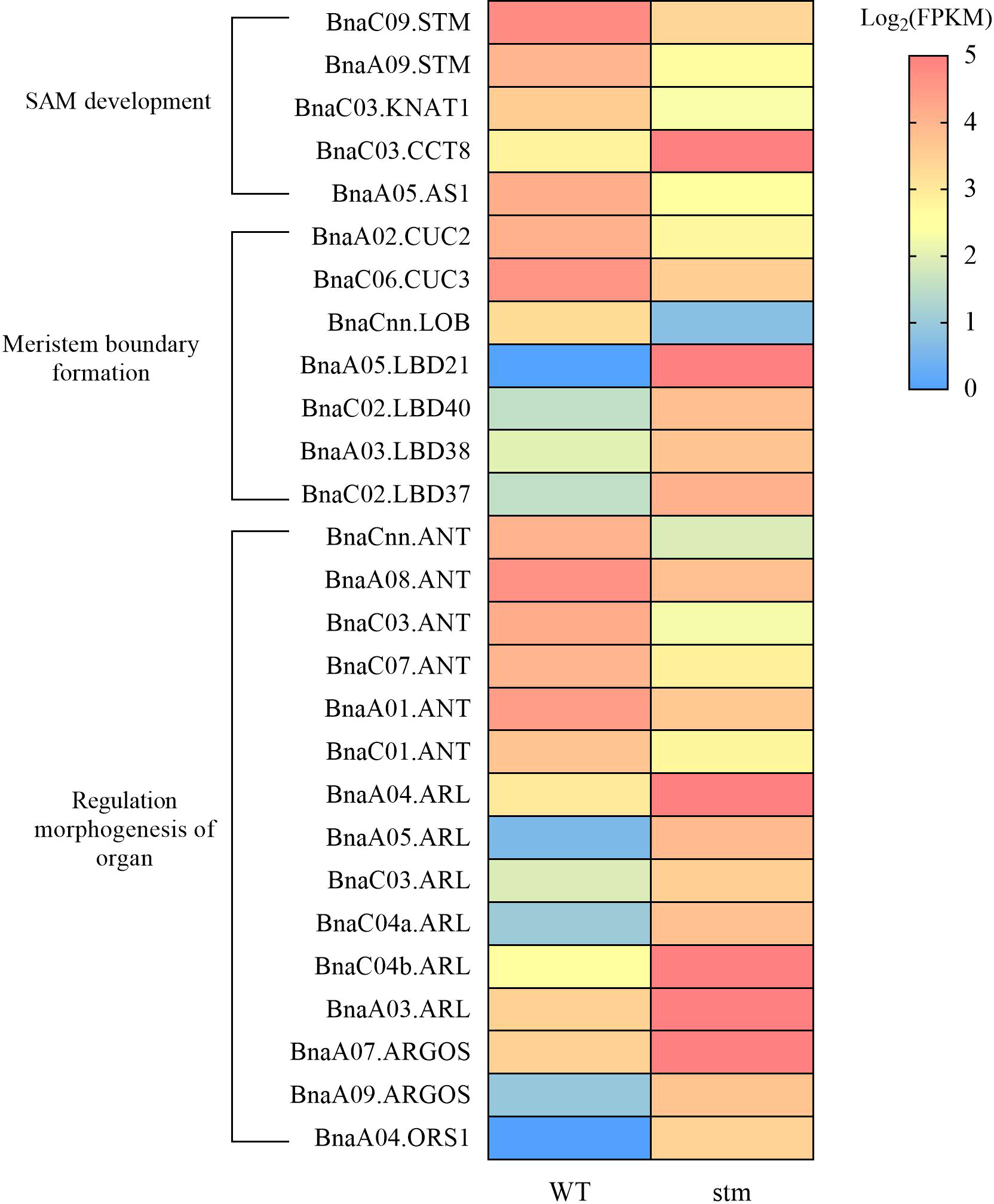
Figure 6 Heatmap showing the expression (FPKM) of important genes related to the development of shoot apical meristem (SAM) in double mutant and WT.
Notably, BnaCUC2 and BnaCUC3 were down-regulated to varying degrees (Figure 6). As we know that CUCs were involved in the formation of the SAM boundary (Kwon et al., 2006), The down-regulation of CUCs expression may lead to cotyledon petiole fusion. In addition, LOB DOMAIN-CONTAINING (LBD) gene family contain LATERAL ORGAN BOUNDARIES (LOB) domain, so LBDs can also regulate the formation of plant lateral organ boundary in plants (Xu et al., 2016). In our transcriptome data, the expression of LBD family genes showed irregular rise and fall, such as BnaLBD40, BnaLBD21, BnaLBD38, and other genes increased significantly, whereas, BnaLOB gene expression decreased significantly.
AP2-LIKE ETHYLENE-RESPONSIVE TRANSCRIPTION FACTOR (ANT) can maintain the meristematic competence of cells and consequently sustains expression of cell cycle regulators during organogenesis, thus controlling the final size of each organ by controlling their cell number (Horstman et al., 2014). AUXIN-REGULATED GENE INVOLVED IN ORGAN SIZE (ARGOS), ARGOS-LIKE PROTEIN (ARL) and ORGAN SIZE RELATED 1 (ORS1) regulate the formation of organs by regulating the expression of ANT (Hu et al., 2003; Hu et al., 2006; Feng et al., 2011). RNA-seq data shows that in the double mutant, the expression of six BnaANT copies is significantly down-regulated, and some copies of BnaARGOS, BnaARL and BnaORS1 are also differentially expressed (Figure 6). Thus, decrease of the genes in meristem boundary formation might be responsible for the fused cotyledon petiole (Figure 3J) and cup-cotyledon phenotype (Figure 3M).
As AtSTM is engaged in CK and GA metabolism (Jasinski et al., 2005; Yanai et al., 2005), we also dive into our transcriptome data for CK and GA pathways, however, to our surprise, although plant hormone signal transduction pathway was significantly enriched in down-regulated gene sets (Figure S9), the expression of related genes which were previously shown to be involved in SAM regulation (Jasinski et al., 2005), such as GA20ox1, did not change. Only BnaARF5 were significantly down-regulated. This is probably the reason why Bnastm double mutant plants could develop to normal plants while in Arabidopsis stm mutant plants had severer defects in plant development (Jasinski et al., 2005).
To verify the reliability of the RNA-seq data, 21 genes in the seedling were selected for qRT-PCR verification analysis. These genes included 12 genes related to SAM development, and 9 were randomly selected DEGs (Table S5). The linear regression analysis showed that the measured correlation coefficient between the two sets of gene transcription levels was very high (R = 0.884; Figure S10), further confirming the reliability of the RNA-seq data.
The results showed that BnaSTM plays an important role in the development of SAM. Its expression can cause changes in the formation of SAM boundaries as well as early organogenesis. These results indicated that BnaSTM has more complex regulatory networks and mechanisms in B. napus.
3.8 Bnastm altered expression of genes in FA and glucosinolate biosynthesis pathways in the seedling but not the of FA and glucosinolate content in the seeds
Interestingly, the expression levels of genes related to fatty acid synthesis and oleic acid synthesis were significantly up-regulated. For instance, one of the three copies of BnaFAD2 and one copies of BnaAAC1 was up-regulated, which are the key genes for fatty acid synthesis (Table S6). In addition, based on the KEGG enrichment analysis, glucosinolate biosynthesis pathway was significantly enriched in both up- and down-regulated gene sets (Figure S9; Table S7). The expression level of genes BnaBCAT4, BnaMAM1, BnaIPMDH1 and BnaIIL1 regulating the side-chain elongation of glucosinolate was significantly down-regulated, and the expression level of BnaSOT18 gene regulating the synthesis of aliphatic and indole glucosinolate core structure was significantly up-regulated.
Fatty acid composition and content, protein content and glucosinolate content are important traits in rapeseed. The composition and content of fatty acids determine the yield and quality of oil, while the content of protein determines the quality of rapeseed meal. Glucosinolates are anti-cancer and antioxidant biochemical compounds that can protect plants from insects and microorganisms (Halkier and Gershenzon, 2006). In B. napus, reducing its content in seeds and increasing its content in leaves and other tissues is also one of the goals of rapeseed breeding. Through phenotypic and genotypic analysis, it was found that aaCC and aaCC mutants had almost no difference from WT at early seedling stage (Figure 3; Table 1), and only the double mutants had fused cotyledons. Therefore, we have reason to believe that both copies of STM in B. napus have redundancy function. Therefore, we only selected mature seeds of aaCC and aacc for the analysis of the fatty acid and glucosinolate contents. To our surprise, neither fatty acid nor glucosinolate contents were changed (Figure 7). The total oil content and the three major unsaturated fatty acids: oleic acid (C18:1), linoleic acid (C18:2) and linolenic acid (C18:3) were not significantly changed compared with WT.
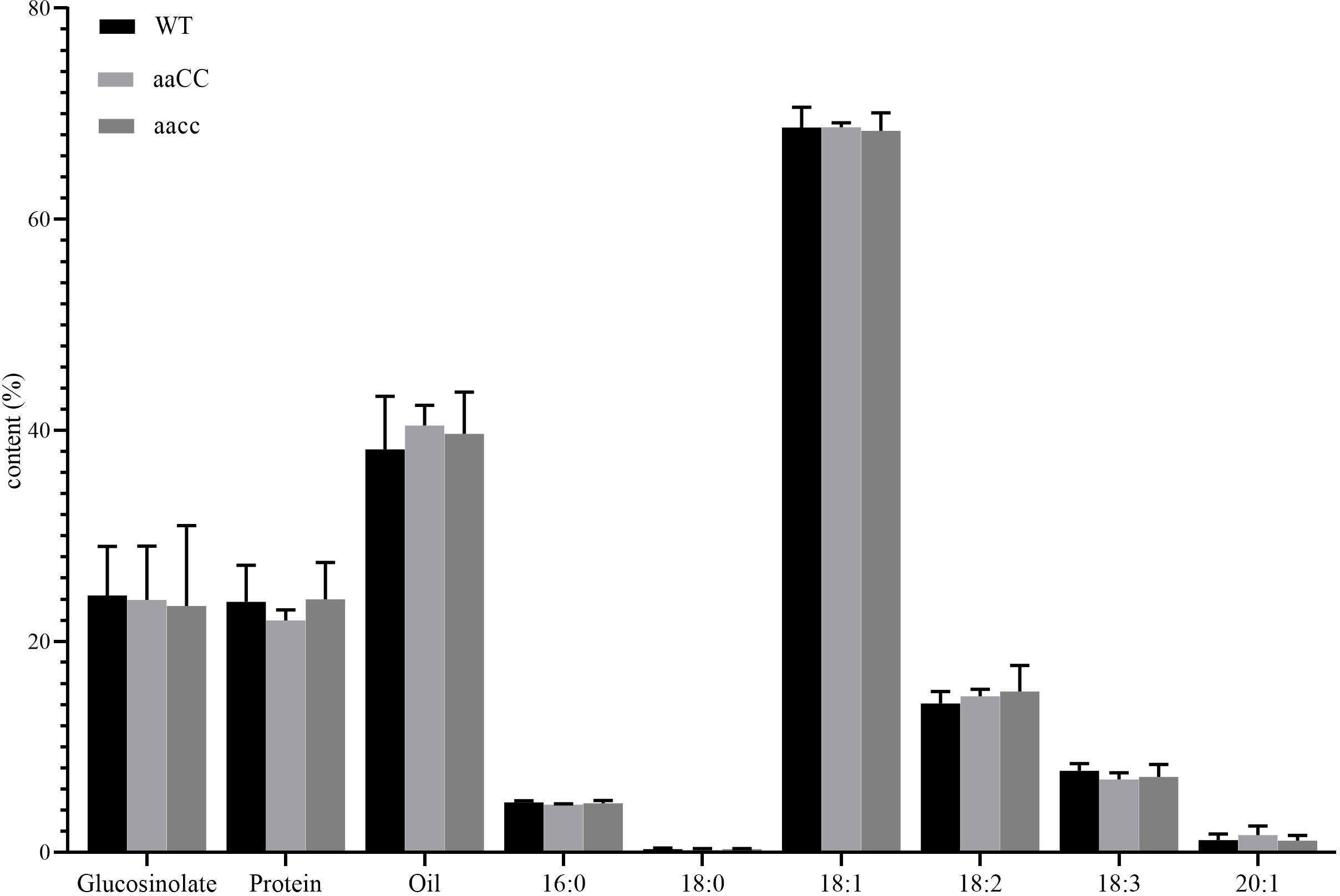
Figure 7 Assay of glucosinolate content, protein content and FA composition in seeds of BnaSTM single (aaCC) and double (aacc) mutants and WT. Three biological replicates were performed, and means ± SE are shown (Student’s t-test).
4 Discussion
The KNOX transcription factor STM is essential during shoot apical meristem development. In Arabidopsis thaliana, STM regulates hormone synthesis and response. It also coordinates the organ formation and differentiation, leaf type, meristem, and the establishment of organ boundary region (Spinelli et al., 2011; Kamiuchi et al., 2014; Balkunde et al., 2017). Therefore, it is also crucial to explore the mechanism of this gene in the important oil crop B. napus.
4.1 Application of CRISPR/Cas9 system in this experiment
Plant genome editing technology depends on plant genetic transformation to a large extent. Compared with other crops, the transformation efficiency of most B. napus is relatively low. For example, Braatz et al. used the Cas9/sgRNA system to conduct gene editing with the spring cultivar Haydn as the transformation recipient, and its first-generation transformation rate was only 0.9% (Braatz et al., 2017). Therefore, this experiment selected a pure B. napus line J9707, which is a good transformation receptor, and the transformation rate is between 66.7% and 92.5% (Yang et al., 2018). At the same time, Hi-tom, a high-throughput sequencing method, is also used to quickly identify genotypes (Yang et al., 2017).
For using CRISPR/Cas9 system, off-target locus check is an important step to perform. According to the results of the editing and off-target identification of other genes in B. napus by the research teams through CRISPR/Cas9 system (Yang et al., 2017; Yang et al., 2018), when the predicted number of base differences between the target locus and the off-target locus is greater than or equal to four, there is no off-target. Moreover, the predicted genes at the off-target locus in this experiment are not related to the development of SAM. Therefore, in the present study, we did not verify the off-target locus by PCR.
What’s more, as an allotetraploid, many genes in B. napus have copy redundancy. The BnaSTM in the present study only has two copies, we used a CRISPR/Cas9 system that can mutate multiple target locus at the same time in B. napus (Yang et al., 2017), which showed good editing efficiency for both copies, and this system was successfully applied to BnaCLV3, which also contained two copies (Yang et al., 2018). However, as for the genes with greater number of copies, it is very difficult to edit all copies at the same time.
4.2 The phenotypes of Bnastm mutant were different from Atstm
In the present study, CRISPR/Cas9 technology was successfully employed to target mutations in BnaSTM. The induced mutations can be stably inherited to progeny. The Bnastm double mutant showed a fused cotyledon petiole phenotype at the seedling stage (Figure 3J), which was similar to the stm-1 (strong mutant allele) in the model plant Arabidopsis (Nidhi et al., 2021). In Arabidopsis, the fusion was restricted to the region from the base to the middle part of the petioles and did not extend to the cotyledon ([]Clark, 1996; Long et al., 1996; Aida et al., 1999; Belles-Boix et al., 2006; Nidhi et al., 2021). However, one of the Bnastm double mutant seedling had a cup-shaped cotyledon phenotype (Figure 3M), which was not observed in stm mutants in Arabidopsis, but in double mutant with KNAT6 and CUC2 (Belles-Boix et al., 2006). This indicates that early boundary regulation of STM in B. napus is more complex.
In addition to the seedling phenotype, Bnastm also had great differences in the SAM, leaves and inflorescence development compared with Atstm. The cytological observation revealed that SAM was not observed in the mature embryo of double mutant (Figure 5A) which was the same as in Arabidopsis (Clark, 1996). During seed germination process, BnaSTM gradually restored SAM after 3 days of germination with no significant difference from WT (Figure 5D). The true leaves which developed later gradually broke wrapped state and resumed normal growth in the late vegetative growth period (Figure 3L). The growth and development of the double mutant in the seedling stage were slightly slower than WT (Figure 3K). In addition to physical oppression, it may also be that SAM formation in Bnastm double mutants delayed compared to the WT one, resulting in delayed expression of genes that regulate stem cell differentiation. The results showed that SAM could develop normally in WT, and that the genes regulating stem cell differentiation could be expressed normally without STM mutation. Surprisingly, in the older stage of growth and development, there was no significant difference from the WT (Figure 4). However, in Arabidopsis. the severe mutant stm-1 in Arabidopsis lack an embryonic shoot meristem and could not produce normal leaves and inflorescence (Clark, 1996; Nidhi et al., 2021), while a weak allele stm-2 could produce leaves and inflorescences that were not indeterminate structures as in WT plants (Clark, 1996). This implies that there may also be functionally redundant genes with BnaSTM in B. napus for functional compensation or BnaSTM has more complex regulatory networks and mechanisms in B. napus compared with A. thaliana.
4.3 BnaSTM is mainly involved in the shoot meristem boundary formation and organogenesis
According to transcriptome results, in the KNOX I gene family, except BnaSTM, only the expression level of BnaKNAT1 was significantly reduced in the double mutant. Research showed that AS1 and AS2 inhibit the expression of KNOX I genes including KNAT1, KNAT2, KNAT6 and STM (Semiarti et al., 2001). The down-regulation of BnaAS1 expression in mutants may promote the up regulation of BnaKNAT1, BnaKNAT2 and BnaKNAT6 expression to compensate for the effect of Bnastm mutation. As the BnaCCT8 functions in transporting the STM protein to maintain the homeostasis of stem cell (Xu et al., 2011), the down-regulating of the transporting of STM by BnaCCT8 is probably the result of the down-regulation of BnaSTM, so that the homeostasis of stem cell was defected.
As we observed the fused cotyledon petiole in the seedling of Bnastm mutants, it was not surprising that genes involved in meristem boundary formation had significant changes in the transcriptome data compared with WT. Bnastm mutations significantly reduced the expression of BnaCUC2 and BnaCUC3, which were known to be involved in the establishment of boundaries (Vroemen et al., 2003). However, the expression of other regulatory boundary genes was significantly increased in mutants, such as BnaLBD family genes. BnaLBD family genes were key regulators of lateral organ boundary set up and played essential roles in integrating developmental changes in response to phytohormone signaling or environmental cues (Xu et al., 2016). Although the specific functions of each gene in the BnaLBD gene family are still unclear, the expression patterns and functional domains of these genes suggest that up-regulation of these genes may compensate for the effects of Bnastm mutation (Clark et al., 1997) (Figure 6).
In addition to boundary formation genes, a sets of organogenesis related genes were significantly changed in Bnastm (Figure 6). ANT regulates cell proliferation and organ growth by maintaining the meristematic competence of cells during organogenesis (Mizukami and Fischer, 2000). ANT affects cell proliferation by regulating the expression of Cyclin D3;1 (CYCD3;1) during organ growth (Hu et al., 2003). The expression of BnaANT decreased significantly (Figure 6), it can be speculated that there may be a certain regulatory mechanism between BnaSTM and BnaANT. When BnaSTM was knocked out, the expression of BnaANT gene was decreased, which together led to the smaller SAM (Figure 5) and true leaf size (Figure 3) compared with WT. Besides, increased expression of BnAGROS, BnARL and BnORS1 was observed. AGROS, ARL and ORS1 contain a conserved OSR motif which regulates organ growth and final organ size by affecting both cell proliferation and expansion, and OSR1 regulates organ growth redundantly with ARGOS and ARL (Feng et al., 2011). In Arabidopsis, overexpression of OSR1 prolonged the expression of ANT but not increase the expression level. Therefore, we hypothesized that in B. napus, the increase of the expression level of BnaARGOS, BnaARL and BnaORS1 was the feedback mechanism of plant, which try to overcome the decrease of the expression of ANT.
Several studies have shown that CLV/WUS signaling pathway is an important pathway to maintain SAM homeostasis. According to previous studies, the inhibitory effect of CLV mutants on stem cell differentiation was weakened, leading to abnormal meristem development in plants and the formation of a multilocular angular phenotype (Clark et al., 1997; Song et al., 2021). It has been reported that CLV3 and WUS are mainly expressed in the inflorescence meristem after bolting in Arabidopsis (Winter et al., 2007), and mainly expressed in flower buds and seeds in Brassica (Liu et al., 2021), and their expression levels are very low, which is consistent with the results in this study that CLV3 and WUS are almost not expressed in WT and stm mutants.
According to reports in Arabidopsis, A ring of AINTEGUMENTA gene expression distinguishes the peripheral domain, from which the cotyledons arise, from the central domain, where the meristem will form. CUCs, STM and KNAT6 are expressed in a stripe across the middle of the embryo between the cotyledon primordia (Barton, 2010). At present, there are few studies on BnaSTM in rapeseed and there is no report on how it is expressed in different regions in SAM, it may be possible to observe the specific expression region of STM in SAM of B. napus by constructing a fluorescent protein vector including STM and help us explain the specific mechanism of STM in B. napus.
4.4 Effect of BnaSTM on the biosynthesis of fatty acids and glucosinolate
Interestingly, the transcriptome data of 7-days-old seedling results showed that the expression of BnaFAD2, BnaFAD3 and BnaACC1 were up-regulated, which was related to fatty acid synthesis. According to a previous study, the ACC1 participates in the cell proliferation and tissue mode in the development of SAM, which can act as a repressor of CK response (Baud et al., 2003). However, although the expression of BnaFAD2 and BnaFAD3 increased, the oil content and oil composition of mature seeds did not change significantly. Because FAD2, FAD3 and other important genes regulating oil content are mainly expressed and play a role in silique and seed at the later stage of plant growth, these genes are differentially expressed in SAM in this experiment, and the expression period is far earlier than the period when seed start to form.
The same is true for genes related to glucosinolate biosynthesis (Figure 7; Table S8). The reason may be that the down-regulation of the expression of BnaBCAT4, as the starting gene regulating glucosinolate biosynthesis, leads to the down-regulation of the expression of genes in the MANs-IPMIs-IPMDHs cycle which regulating amino acid chain elongation of glucosinolate biosynthesis (Gao et al., 2014). BnaSOT18, as the key gene regulating the biosynthesis of aliphatic and indole glucosinolate, the up-regulation of its expression compensates for the effect of down-regulation of BnaBCAT4 on glucosinolate biosynthesis.
Furthermore, our transcriptome data was acquired at the seedling stage, while the fatty acid content and the glucosinolate content were measured in the mature seeds. As in B. napus, we only observed the phenotype in seedling stage (Figures 3, 4), but not in the adult plants (Figure 5), it could be speculated that BnaSTM is mainly function during the early organ formation but not in late plant development in B. napus.
In all, STM is an important gene regulating SAM in B. napus, have many unexplored functions. Further studies on identifying its function can help to understand the growth process of B. napus more clearly as well as a better understanding of other related genes.
Data availability statement
The datasets presented in this study can be found in online repositories. The names of the repository/repositories and accession number(s) can be found in the article/Supplementary Material.
Author contributions
YZ and CF conducted the study. YZ and CF designed the experiments. HL and XW performed the experiments. KY performed the bioinformatic analysis. XW and HL assisted in the material sampling. KY wrote the manuscript. HH, HL, OA, and CF helped in the revision of the manuscript. CF supervised the study. All authors contributed to the article and approved the submitted version.
Funding
The study was financially supported by China Agriculture Research System (CARS-12), the National Natural Science Foundation of China (32172021, 31371240, 31671279) and the National Key Research and Development Program of China (2020YFD1000902, 2022YFD1200401).
Conflict of interest
The authors declare that the research was conducted in the absence of any commercial or financial relationships that could be construed as a potential conflict of interest.
Publisher’s note
All claims expressed in this article are solely those of the authors and do not necessarily represent those of their affiliated organizations, or those of the publisher, the editors and the reviewers. Any product that may be evaluated in this article, or claim that may be made by its manufacturer, is not guaranteed or endorsed by the publisher.
Supplementary material
The Supplementary Material for this article can be found online at: https://www.frontiersin.org/articles/10.3389/fpls.2023.1042430/full#supplementary-material
References
Aida, M., Ishida, T., Tasaka, M. (1999). Shoot apical meristem and cotyledon formation during arabidopsis embryogenesis: interaction among the CUP-SHAPED COTYLEDON and SHOOT MERISTEMLESS genes. Development. 126 (8), 1563–1570. doi: 10.1242/dev.126.8.1563
Balkunde, R., Kitagawa, M., Xu, X. M., Wang, J., Jackson, D. (2017). SHOOT MERISTEMLESS trafficking controls axillary meristem formation, meristem size, and organ boundaries in arabidopsis. Plant J. 90 (3), 435–446. doi: 10.1111/tpj.13504
Barton, M. K. (2010). Twenty years on: The inner workings of the shoot apical meristem, a developmental dynamo. Dev. Biol. 341 (1), 95–113. doi: 10.1016/j.ydbio.2009.11.029
Baud, S., Guyon, V., Kronenberger, J., Wuillème, V., Miquel, M., Caboche, M., et al. (2003). Multifunctional acetyl-CoA carboxylase 1 is essential for very long chain fatty acid elongation and embryo development in arabidopsis. Plant J. 33 (1), 75–86. doi: 10.1046/j.1365-313x.2003.016010.x
Belles-Boix, E., Hamant, O., Witiak, S. M., Morin, H., Traas J Pautot, V. (2006). KNAT6: an arabidopsis homeobox gene involved in meristem activity and organ separation. Plant Cell. 18 (8), 1900–1907. doi: 10.1105/tpc.106.041988
Boscá, S., Knauer, S., Laux, T. (2011). Embryonic development in Arabidopsis thaliana: from the zygote division to the shoot meristem. Front. Plant Sci. 2. doi: 10.3389/fpls.2011.00093
Braatz, J., Harloff, H. J., Mascher, M., Stein, N., Himmelbach, A., Jung, C. (2017). CRISPR-Cas9 targeted mutagenesis leads to simultaneous modification of different homoeologous gene copies in polyploid oilseed rape (Brassica napus). Plant Physiol. 174 (2), 935–942. doi: 10.1104/pp.17.00426
Brand, U., Grünewald, M., Hobe, M., Simon, R. (2002). Regulation of CLV3 expression by two homeobox genes in arabidopsis. Plant Physiol. 129 (2), 565–575. doi: 10.1104/pp.001867
Bureau, M., Rast, M. I., Illmer J Simon, R. (2010). JAGGED LATERAL ORGAN (JLO) controls auxin-dependent patterning during development of the arabidopsis embryo and root. Plant Mol. Biol. 74 (4-5), 479–491. doi: 10.1007/s11103-010-9688-2
Chaplin, A. J. (1999). The use of histological techniques for the demonstration of ion exchange resins. J. Clin. Pathol. 52 (10), 776. doi: 10.1136/jcp.52.10.776
Clark, SE., Jacobsen, SE., Levin, JZ., Meyerowitz, EM. (1996). The CLAVATA and SHOOT MERISTEMLESS loci competitively regulate meristem activity in arabidopsis. Development 122(5), 1567-1575. doi: 10.1242/dev.122.5.1567
Clark, S. E., Williams, R. W., Meyerowitz, E. M. (1997). The CLAVATA1 gene encodes a putative receptor kinase that controls shoot and floral meristem size in arabidopsis. Cell. 89 (4), 575–585. doi: 10.1016/s0092-8674(00)80239-1
Cole, M., Nolte C Werr, W. (2006). Nuclear import of the transcription factor SHOOT MERISTEMLESS depends on heterodimerization with BLH proteins expressed in discrete sub-domains of the shoot apical meristem of arabidopsis thaliana. Nucleic Acids Res. 34 (4), 1281–1292. doi: 10.1093/nar/gkl016
Doebley, J. (2004). The genetics of maize evolution. Annu. Rev. Genet. 38 (1), 37–591792. doi: 10.1146/annurev.genet.38.072902.092425
Elhiti, M., Wally, O. S., Belmonte, M. F., Chan, A., Cao, Y., Xiang, D., et al. (2013). Gene expression analysis in microdissected shoot meristems of Brassica napus microspore-derived embryos with altered SHOOT MERISTEMLESS levels. Planta. 237 (4), 1065–1082. doi: 10.1007/s00425-012-1814-8
Fan, C., Wu, Y., Yang, Q., Yang, Y., Meng, Q. W., Zhang, K. Q., et al. (2014). A novel single-nucleotide mutation in a CLAVATA3 gene homolog controls a multilocular silique trait in Brassica rapa l. Mol. Plant 7 (12), 1788. doi: 10.1093/mp/ssu090
Feng, G., Qin, Z., Yan, J., Zhang, X., Hu, Y. (2011). Arabidopsis ORGAN SIZE RELATED1 regulates organ growth and final organ size in orchestration with ARGOS and ARL. New Phytol. 191 (3), 635–646. doi: 10.1111/j.1469-8137.2011.03710.x
Gallois, J. L., Woodward, C., Reddy, G. V., Sablowski, R. (2002). Combined SHOOT MERISTEMLESS and WUSCHEL trigger ectopic organogenesis in arabidopsis. Development. 129 (13), 3207–3217. doi: 10.1242/dev.129.13.3207
Gao, J., Yu, X., Ma, F., Li, J. (2014). RNA-Seq analysis of transcriptome and glucosinolate metabolism in seeds and sprouts of broccoli (Brassica oleracea var. italic). PloS One 9 (2), e88804. doi: 10.1371/journal.pone.0088804
Guo, M., Thomas, J., Collins G Timmermans, M. C. P. (2008). Direct repression of KNOX loci by the ASYMMETRIC LEAVES1 complex of arabidopsis. Plant Cell. 20 (1), 48–58. doi: 10.1105/tpc.107.056127
Halkier, B. A., Gershenzon, J. (2006). Biology and biochemistry of glucosinolates. Annu. Rev. Plant Biol. 57, 303–333. doi: 10.1146/annurev.arplant.57.032905.105
Hay, A., Tsiantis, M. (2010). KNOX genes: versatile regulators of plant development and diversity. Development. 137 (19), 3153–3165. doi: 10.1242/dev.030049
Horstman, A., Willemsen, V., Boutilier K Heidstra, R. (2014). AINTEGUMENTA-LIKE proteins: hubs in a plethora of networks. Trends Plant Sci. 19 (3), 146–157. doi: 10.1016/j.tplants.2013.10.010
Hu, Y., Poh, H. M., Chua, N. H. (2006). The arabidopsis ARGOS-LIKE gene regulates cell expansion during organ growth. Plant J. 47 (1), 1–9. doi: 10.1111/j.1365-313X.2006.02750.x
Hu, Y., Xie, Q., Chua, N. H. (2003). The arabidopsis auxin-inducible gene ARGOS controls lateral organ size. Plant Cell. 15 (9), 1951–1961. doi: 10.1105/tpc.013557
Jasinski, S., Piazza, P., Craft, J., Hay, A., Woolley, L., Rieu, I., et al. (2005). KNOX action in arabidopsis is mediated by coordinate regulation of cytokinin and gibberellin activities. Curr. Biol. 15 (17), 1560–1565. doi: 10.1016/j.cub.2005.07.023
Kamiuchi, Y., Yamamoto, K., Furutani, M., Tasaka M Aida, M. (2014). The CUC1, and CUC2 genes promote carpel margin meristem formation during arabidopsis gynoecium development. Front. Plant Sci. 5. doi: 10.3389/fpls.2014.00165
Kierzkowski, D., Runions, A., Vuolo, F., Strauss, S., Lymbouridou, R., Routier-Kierzkowska, A.-L., et al. (2019). A growth-based framework for leaf shape development and diversity. Cell. 177 (6), 1405–1418. doi: 10.1016/j.cell.2019.05.011
Kumaran, M. K., Bowman, J. L., Sundaresan, V. (2002). YABBY polarity genes mediate the repression of KNOX homeobox genes in arabidopsis. Plant Cell. 14 (11), 2761–2770. doi: 10.1105/tpc.004911
Kwon, C. S., Hibara, K., Pfluger, J., Bezhani, S., Metha, H., Aida, M., et al. (2006). A role for chromatin remodeling in regulation of CUC gene expression in the arabidopsis cotyledon boundary. Development. 133 (16), 3223–3230. doi: 10.1242/dev.02508
Lei, Y., Lu, L., Liu, H. Y., Li, S., Xing, F., Chen, L. L. (2014). CRISPR-p: a web tool for synthetic single-guide RNA design of CRISPR-system in plants. Mol. Plant 7 (9), 1494–1496. doi: 10.1093/mp/ssu044
Lenhard, M., Jürgens, G., Laux, T. (2002). The WUSCHEL and SHOOTMERISTEMLESS genes fulfil complementary roles in arabidopsis shoot meristem regulation. Development. 129 (13), 3195–3206. doi: 10.1242/dev.129.13.3195
Liu, L., Li, C., Song, S., Teo, Z. W. N., Shen, L., Wang, Y., et al. (2018). FTIP-dependent STM trafficking regulates shoot meristem development in arabidopsis. Cell Rep. 23 (6), 1879–1890. doi: 10.1016/j.celrep.2018.04.033
Liu, D., Yu, L., Wei, L., Yu, P., Wang, J., Zhao, H., et al. (2021). BnTIR: An online transcriptome platform for exploring RNA-seq libraries for oil crop brassica napus. Plant Biotechnol. J. 19 (10), 1895–1897. doi: 10.1111/pbi.13665
Long, J. A., Moan, E. I., Medford, J. I., Barton, M. K. (1996). A member of the KNOTTED class of homeodomain proteins encoded by the STM gene of arabidopsis. Nature. 379 (6560), 66–69. doi: 10.1038/379066a0
Lu, H., Wu, H., Zhu, G., Yin, C., Zhao, L., Wen, J., et al. (2022). Identification and fine mapping of the candidate gene controlling multi-inflorescence in brassica napus. Int. J. Mol. Sci. 23 (13), 7244. doi: 10.3390/ijms23137244
Ma, X., Zhang, Q., Zhu, Q., Liu, W., Chen, Y., Qiu, R., et al. (2015). A robust CRISPR/Cas9 system for convenient, high-efficiency multiplex genome editing in monocot and dicot plants. Mol. Plant 8 (8), 1274–1284. doi: 10.1016/j.molp.2015.04.007
Mizukami, Y., Fischer, R. L. (2000). Plant organ size control: AINTEGUMENTA regulates growth and cell numbers during organogenesis. Proc. Natl. Acad. Sci. U S A. 97 (2), 942–947. doi: 10.1073/pnas.97.2.942
Nidhi, S., Preciado, J., Tie, L. (2021). Knox Homologs SHOOT MERISTEMLESS (STM) and KNAT6 are epistatic to CLAVATA3 (CLV3) during shoot meristem development in arabidopsis thaliana. Mol. Biol. Rep. 48 (9), 6291–6302. doi: 10.1007/s11033-021-06622-4
Ohmori, Y., Tanaka, W., Kojima, M., Sakakibara, H., Hirano, H. (2013). WUSCHEL-RELATED HOMEOBOX4 is involved in meristem maintenance and is negatively regulated by the CLE gene FCP1 in rice. Plant Cell. 25 (1), 229–241. doi: 10.1105/tpc.112.103432
Perales, M., Rodriguez, K., Snipes, S., Yadav, R. K., Diaz-Mendoza M Reddy, G. V. (2016). Threshold-dependent transcriptional discrimination underlies stem cell homeostasis. Proc. Natl. Acad. Sci. U S A. 113 (41), E6298–EE306. doi: 10.1073/pnas.1607669113
Piazza, P., Bailey, C., Cartolano, M., Krieger, J., Cao, J., Ossowski, S., et al. (2010). Arabidopsis thaliana leaf form evolved via loss of KNOX expression in leaves in association with a selective sweep. Curr. Biol. 20 (24), 2223–2228. doi: 10.1016/j.cub.2010.11.037
Ragni, L., Belles-Boix, E., Gunl M Pautot, V. (2008). Interaction of KNAT6 and KNAT2 with BREVIPEDICELLUS and PENNYWISE in arabidopsis inflorescences. Plant Cell. 20 (4), 888–900. doi: 10.1105/tpc.108.058230
Rast MI Simon, R. (2012). Arabidopsis JAGGED LATERAL ORGANS acts with ASYMMETRIC LEAVES2 to coordinate KNOX and PIN expression in shoot and root meristems. Plant Cell. 24 (7), 2917–2933. doi: 10.1105/tpc.112.099978
Roth, O., Alvarez, J. P., Levy, M., Bowman, J. L., Ori, N., Shani, E. (2018). The KNOXI transcription factor SHOOT MERISTEMLESS regulates floral gate in arabidopsis. Plant Cell. 30 (6), 1309–1321. doi: 10.1105/tpc.18.00222
Schoof, H., Lenhard, M., Haecker, A., Mayer, K. F. X., Jürgens G Laux, T. (2000). The stem cell population of arabidopsis shoot meristems is maintained by a regulatory loop between the CLAVATA and WUSCHEL genes. Cell. 100 (6), 635–644. doi: 10.1016/s0092-8674(00)80700-x
Scofield, S., Dewitte, W., Nieuwland, J., Murray, J. A. H. (2013). The arabidopsis homeobox gene SHOOT MERISTEMLESS has cellular and meristem-organisational roles with differential requirements for cytokinin and CYCD3 activity. Plant J. 75 (1), 53–66. doi: 10.1111/tpj.12198
Scofield, S., Murison, A., Jones, A., Fozard, J., Aida, M., Band, L. R., et al. (2018). Coordination of meristem and boundary functions by transcription factors in the SHOOT MERISTEMLESS regulatory network. Development. 145 (9), 157081. doi: 10.1242/dev.157081
Scofield, S., Murray, J. A. (2006). KNOX gene function in plant stem cell niches. Plant Mol. Biol. 60 (6), 929–946. doi: 10.1007/s11103-005-4478-y
Semiarti, E., Ueno, Y., Tsukaya, H., Iwakawa, H., Machida, C., Machida, Y. (2001). The ASYMMETRIC LEAVES2 gene of Arabidopsis thaliana regulates formation of a symmetric lamina, establishment of venation and repression of meristem-related homeobox genes in leaves. Development. 128 (10), 1771–1783. doi: 10.1242/dev.128.10.1771
Shahid, M., Cai, G., Zu, F., Zhaoi, Q., Qasim, MU., Hong, Y., et al. (2019). Comparative transcriptome analysis of developing seeds and silique wall reveals dynamic transcription networks for effective oil production in Brassica napus l. Int. J. Mol. Sci. doi: 10.3390/ijms20081982
Song, S., Chen, Y., Liu, L., Wang, Y., Bao, S., Zhou, X., et al. (2017). OsFTIP1-mediated regulation of florigen transport in rice is negatively regulated by the ubiquitin-like domain kinase OsUbDKγ4. Plant Cell 29 (3), 491–507. doi: 10.1105/tpc.16.00728
Song, X. F., Hou, X. L., Liu, C. M. (2021). CLE peptides: critical regulators for stem cell maintenance in plants. Planta. 255 (1), 5. doi: 10.1007/s00425-021-03791-1
Spinelli, S. V., Martin, A. P., Viola, I. L., Gonzalez, D. H., Palatnik, J. F. (2011). A mechanistic link between STM and CUC1 during arabidopsis development. Plant Physiol. 156 (4), 1894–1904. doi: 10.1104/pp.111.177709
Su, Y. H., Zhou, C., Li, Y. J., Yu, Y., Tang, L. P., Zhang, W. J., et al. (2020). Integration of pluripotency pathways regulates stem cell maintenance in the arabidopsis shoot meristem. Proc. Natl. Acad. Sci. U S A. 117 (36), 22561–22571. doi: 10.1073/pnas.2015248117
Takada, S., Hibara, K., Ishida, T., Tasaka, M. (2001). The CUP-SHAPED COTYLEDON1 gene of arabidopsis regulates shoot apical meristem formation. Development. 128 (7), 1127–1135. doi: 10.1242/dev.128.7.1127
USDA ERS. (2021). Available at: https://www.ers.usda.gov/data-products/oil-crops-yearbook/.
Venglat, S., Dumonceaux, T., Rozwadowski, K., Parnell, L., Babic, V., Keller, W., et al. (2002). The homeobox gene BREVIPEDICELLUS is a key regulator of inflorescence architecture in arabidopsis. Proc. Natl. Acad. Sci. U S A. 99 (7), 4730–4735. doi: 10.1073/pnas.072626099
Vroemen, C. W., Mordhorst, A. P., Albrecht, C., Kwaaitaal, M. A., de Vries, S. C. (2003). The CUP-SHAPED COTYLEDON3 gene is required for boundary and shoot meristem formation in arabidopsis. Plant Cell. 15 (7), 1563–1577. doi: 10.1105/tpc.012203
Winter, D., Vinegar, B., Nahal, H., Ammar, R., Wilson, G. V., Provart, N. J. (2007). An “Electronic fluorescent pictograph” browser for exploring and analyzing large-scale biological data sets. PloS One 2 (8), e718. doi: 10.1371/journal.pone.0000718
Xu, C., Luo, F., Hochholdinger, F. (2016). LOB domain proteins: beyond lateral organ boundaries. Trends Plant Sci. 21 (2), 159–167. doi: 10.1016/j.tplants.2015.10.010
Xu, X., Wang, J., Xuan, Z., Xuan, Z., Goldshmidt, A., Borrill, P., et al. (2011). Chaperonins facilitate KNOTTED1 cell-to-cell trafficking and stem cell function. Science. 333 (6046), 1141–1144. doi: 10.1126/science.1205727
Xue, Z., Liu, L., Zhang, C. (2020). Regulation of shoot apical meristem and axillary meristem development in plants. Int. J. Mol. Sci. 21 (8), 2917. doi: 10.3390/ijms21082917
Yanai, O., Shani, E., Dolezal, K., Tarkowski, P., Sablowski, R., Sandberg, G., et al. (2005). Arabidopsis KNOXI proteins activate cytokinin biosynthesis. Curr. Biol. 15 (17), 1566–1571. doi: 10.1016/j.cub.2005.07.060
Yang, H., Wu, J. J., Tang, T., Liu, KD., Dai, C.. (2017). CRISPR/Cas9-mediated genome editing efficiently creates specific mutations at multiple loci using one sgRNA in brassica napus. Sci. Rep. 7 (1), 4877. doi: 10.1038/s41598-017-07871-9
Yang, Y., Zhu, K., Li, H., Han, S., Meng, Q., Khan, S. U., et al. (2018). Precise editing of CLAVATA genes in Brassica napus l. regulates multilocular silique development. Plant Biotechnol. J. 16 (7), 1322–1335. doi: 10.1111/pbi.12872
Keywords: Brassica napus L., CRISPR/Cas9, shoot apical meristem (SAM), BnaSTM, fused cotyledon petiole
Citation: Yu K, Li H, Wu X, Amoo O, He H, Fan C and Zhou Y (2023) Targeted mutagenesis of BnaSTM leads to abnormal shoot apex development and cotyledon petiole fusion at the seedling stage in Brassica napus L.. Front. Plant Sci. 14:1042430. doi: 10.3389/fpls.2023.1042430
Received: 12 September 2022; Accepted: 30 January 2023;
Published: 14 February 2023.
Edited by:
Nazim Hussain, Independent Researcher, Sharjah, United Arab EmiratesReviewed by:
Helene S. Robert Boisivon, Central European Institute of Technology (CEITEC), CzechiaJinjin Jiang, Yangzhou University, China
Copyright © 2023 Yu, Li, Wu, Amoo, He, Fan and Zhou. This is an open-access article distributed under the terms of the Creative Commons Attribution License (CC BY). The use, distribution or reproduction in other forums is permitted, provided the original author(s) and the copyright owner(s) are credited and that the original publication in this journal is cited, in accordance with accepted academic practice. No use, distribution or reproduction is permitted which does not comply with these terms.
*Correspondence: Hanzi He, aHpoZUBtYWlsLmh6YXUuZWR1LmNu; Chuchuan Fan, ZmFuY2h1Y2h1YW5AbWFpbC5oemF1LmVkdS5jbg==
†These authors have contributed equally to this work