- 1Molecular Population Genetics and Breeding Group, Temasek Life Sciences Laboratory, 1 Research Link, National University of Singapore, Singapore, Singapore
- 2Department of Biological Sciences, National University of Singapore, Singapore, Singapore
Drought stress is a major environmental hazard. Stomatal development is highly responsive to abiotic stress and has been used as a cellular marker for drought-tolerant crop selection. C3 and C4 crops have evolved into different photosynthetic systems and physiological responses to water deficits. The genome sequences of maize, sorghum, and sugarcane make it possible to explore the association of the stomatal response to drought stress with the evolution of the key stomatal regulators. In this study, phylogenic analysis, gene expression analysis and stomatal assay under drought stress were used to investigate the drought tolerance of C3 and C4 plants. Our data shows that C3 and C4 plants exhibit different drought responses at the cellular level. Drought represses the growth and stomatal development of C3 crops but has little effect on that of C4 plants. In addition, stomatal development is unresponsive to drought in drought-tolerant C3 crops but is repressed in drought-tolerant C4 plants. The different developmental responses to drought in C3 and C4 plants might be associated with the divergent expression of their SPEECHLESS genes. In particular, C4 crops have evolved to generate multiple SPEECHLESS homologs with different genetic structure and expression levels. Our research provides not only molecular evidence that supports the evolutionary history of C4 from C3 plants but also a possible molecular model that controls the cellular response to abiotic stress in C3 and C4 crops.
Introduction
Drought stress is the most critical environmental threat to global food security. The loss in crop yield caused by drought stress is larger than all biologically caused losses combined (Gupta et al., 2020). To adapt to water scarcity in soil, plants have evolved strategies to prevent water loss and maintain their key biological processes (Basu et al., 2016). During drought stress, roots display hydro-morphological changes by adjusting the lateral root emergence to soil with higher water content (Dinneny, 2019). This process is mediated by the EXOCYST SUBUNIT EXO70 FAMILY PROTEIN A3 (EXO70A3) via regulating the homeostasis of the auxin efflux carrier PINFORMED 4 (PIN4) in root tips (Ogura et al., 2019).
Stomata are valve-like openings found in the plant epidermis. The regulation of stomatal development and movement is important for plants to defend against dehydration (Lee and Bergmann, 2019). Stomatal lineage transitions and cell divisions are fine-tuned by three basic helix-loop-helix (bHLH) transcription factors: SPEECHLESS (SPCH) (Macalister et al., 2007), MUTE (Pillitteri et al., 2007) and FAMA (Ohashi-Ito and Bergmann, 2006) and their heterodimer SCREAM1 (Kanaoka et al., 2008). Among them, the expression and regulation of SPCH are highly responsive to the environment, which leads to the stomatal developmental response (Lee and Bergmann, 2019). High temperature represses the expression of SPCH by regulating the expression of PHYTOCHROME-INTERACTING FACTOR 4 (PIF4) at the transcriptional level (Lau et al., 2018). In Arabidopsis, drought induces the activity of the mitogen-activated protein kinase (MAPK) cascade to destabilize the expression of SPCH at the protein level, resulting in a reduction of stomatal density (Kumari et al., 2014). This has been used in the bioengineering of drought-tolerant crops. The overexpression of the extracellular secreted peptide EPIDERMAL PATTERNING FACTOR 2 (EPF2) enhances the drought tolerance of rice by activating the MAPK cascade (Hepworth et al., 2015). A recent study showed that SnRK2 kinases of the ABA signaling pathway directly phosphorylated SPCH in a drought-dependent manner, resulting in the change of stomatal production in response to drought stress (Yang et al., 2022).
Plants have evolved specific adaptation mechanisms to survive short- and long-term drought stresses. Hormones, microRNAs and other transcriptional factors are crosslinked with stomatal plasticity in response to abiotic stresses (Han et al., 2021). Drought stimulates the accumulation of the plant hormone abscisic acid (ABA), which mediates the signal crosstalk with other pathways, such as BRASSINOSTEROIDS pathway during drought stress (Song et al., 2016). The activation of ABA-responding genes, such as SNF1-RELATED KINASE 2 (SnRK2.2), represses the stomatal development and induces stomatal closure (Chater et al., 2014). miR156 mediates stomata behavior under drought stress via ABA-dependent accumulation of strigolactones (Visentin et al., 2020). Stomatal movement is also required for drought tolerance and pathogen defense, which are mainly regulated by reactive oxygen species (ROS) and ABA-signaling (Qi et al., 2018). Many transcriptional factors are involved in the signaling pathways. Under a short-term or initial stage of drought stress, plants activate MYB60 expression and promote root growth to intake more water. By contrast, a long-term or severe drought stress repressed the MYB60 expression, resulting in root growth inhibition and stomatal closure to prevent water loss (Oh et al., 2011). Other key TFs, including AP2/ERF, bZIP, WRKY, YABBY and NAC, are also involved in stomata-dependent drought tolerance (Hussain et al., 2021).
C4 species are essential to the tropical ecosystems (Still et al., 2003) and important agricultural crops (e.g. maize, sugarcane, sorghum). C4 photosynthesis is a marvelous functional evolution for plants. It increases the photosynthetic efficiency at high temperatures, drought and low CO2 levels through separating the carbon fixation from Ribulose-1,5-bisphosphate carboxylase-oxygenase (RuBisCO) and concentrating CO2 around RuBisCO (Sharwood et al., 2016). Dicot C4 plants originated in arid areas, indicating the effects of heat, drought, and salinity as important forces in promoting C4 evolution (Sage, 2004). C4 plants exhibit lower stomatal conductance, which is correlated with their higher water and nitrogen use efficiency compared to C3 species (Taylor et al., 2010). Comparative genomic studies reveal that the evolution of C4 plants depends on whole-genome and individual gene duplication (Wang et al., 2009; Van Den Bergh et al., 2014). The C4 gene homologs may have different adaptive evolution and duplicability (Wang et al., 2009). Interestingly, the rice phosphoenolpyruvate carboxylase gene undergoes rapid evolution and shows C4-like pattern (Wang et al., 2009).
Stomatal development and their response to osmotic stresses are largely studied in C3 plants. Some tropical-grown crops, such as oil palm, exhibit higher salt tolerance due to the divergent regulation of the expression of SPCH (Song et al., 2022). However, the molecular mechanism of how stomata respond to drought stress in C4 plants and the evolutionary divergence of the stomatal developmental regulators are largely unknown. In this study, by using a comparative genomic approach combined with physiological and molecular analysis, we investigated the stomatal response to drought, and the divergent genetic structure and expression of stomatal genes in major C3 and C4 crops. Our findings will facilitate the understanding of the molecular basis of the different drought tolerance and response in C3 and C4 plants.
Material and methods
Plant growth and drought challenge
Arabidopsis col-0 seeds were transferred onto sterilized soil and were kept in a darkroom at 4°C for 3-days. Seedings were germinated and grown in a plant growth chamber at 22°C with 60% relative humidity under long-day conditions (16 h light/8 h dark) at a light intensity of 100 μmol m–2 s–1. For the drought assay, 7 dpg (days post germination) seedlings were either continually watered (control group) or not watered (drought group) for another 7 days (14 dpg with 7 das (days after stress)). Cotyledons from over 20 seedlings were used for stomatal analysis.
Two-year-old oil palm (Elaeis guineensis) seedlings, one-year-old sugarcane (saccharum spontaneum) and sorghum (Sorghum bicolor) plants were grown in a greenhouse with natural tropical environment. Samples from the control group were watered daily while samples from the drought stress group were not watered for 14 days. Over 20 fresh rosette leaves from more than 4 seedlings of each group were used for stomatal analysis. Drought-tolerant oil palms were screened in our previous study (Wang et al., 2020). Drought-tolerant sugarcanes were screened during the drought challenge in the green house.
The seeds of japonica rice (Oryza sativa) were geminated and grown in a petri dish with 20 mL water in the plant growth chamber with a light/dark cycle of 12 h 28°C/12 h 26°C. At 14 dpg, seedlings were transferred into sterilized soil. For drought and stomatal assay, 14 dpg rice seedlings were transferred into the pots with either wet soil (control group) or dry soil (drought group) for another 7 days (21 dpg with 7 das). 21 dpg seedlings were used for stomatal analysis. Over 20 first leaves from more than 20 rice seedlings of each group were used for analysis.
Stomatal assay
Freshly collected leaves were cleared in a 7:1 ethanol:acetic acid buffer overnight and mounted in a 8:2:1 chloral hydrate: water: glycerol clearing buffer for 24 hours. The abaxial leaf epidermis of the leaf slides was captured at 20 × on a Leica DM2500 microscope with a differential contrast interference (DIC) channel. Two images at either 0.25 mm2 or 0.0625 mm2 were captured per leaf from the central regions. The stomatal density was counted by built-in tool the ‘cell counter’ in ImageJ (NIH, USA).
Constructing phylogenetic trees and analyzing protein structure
The current annotation of the protein sequences of sugarcane is still poor. To identify as many homologs of stomatal regulators in sugarcane as possible, the known protein sequences of the three stomatal regulating genes SPCH, MUTE and FAMA of some C3 and C4 plants were used as probe for tBLASTn (McGinnis and Madden, 2004) with a minimum p-value of 1e-30. These C3 and C4 plants are Arabidopsis (At, Arabidopsis thaliana), Oil palm (Eg, Elaeis guineensis), potato (St, Solanum tuberosum), grape (Vv, Vitis vinifera), japonica rice (Os.j, Oryza sativa), soybean (Gm, Glycine max), tomato (Sl, Solanum lycopersicum), maize (Zm, Zea mays), sugarcane (Ss, saccharum spontaneum) and sorghum (Sb, Sorghum bicolor). After which, the homolog candidates were filtered and annotated by BLASTn (McGinnis and Madden, 2004) against the standard nucleotide database. The filtered CDS sequences of the homologs were aligned with the sugarcane proteome reference (Zhang et al., 2018). The validated protein sequences were aligned using the built-in ClustalW (Hung and Weng, 2016) in MEGA-X software (Kumar et al., 2018) by default settings. The protein FLOWERING LOCUS T (FT) (Turck et al., 2008) was used as the outgroup reference. The accession IDs of the protein sequences were listed in Supplementary Table 1. A phylogenetic tree was constructed by using the maximum likelihood method (Strimmer and Von Haeseler, 1996) with 100 bootstraps via MEGA-X software (Kumar et al., 2018). The protein structure was reconstructed by the ColabFold software (Mirdita et al., 2022) based on AlphaFold (Jumper et al., 2021) using the default settings.
RNA extraction, cDNA synthesis and Q-PCR
Total RNA from leaves was extracted using the RNeasy Plant Mini Kit (Qiagen, Germany). RNA quality and quantity were assessed using a previously described method (Song et al., 2022). cDNA was synthesized using M-MLV reverse transcriptase (Promega, USA) following the manufacturer’s protocol. RT-qPCR was performed in a CFX96 touch deep well real time PCR System (Bio-Rad, USA) with the program in a previous study (Liu et al., 2020). RT-qPCR was used to examine the expression of the stomatal regulating genes SPCH, MUTE and FAMA from Saccharum spontaneum, Sorghum bicolor and Oryza sativa for qPCR. β-TUBLIN genes were used as an internal control to normalize the relative expression of genes. A standard cycling program was used for qPCR: initial denaturation at 94°C for 2 min, followed by 40x cycles of denaturation at 94°C for 15s and annealing at 65°C for 1 min. The primers used for Q-PCR are listed in Supplementary Table 2.
Results
Stomatal development and response to drought stress in C3 and C4 plants
As monocots, C3 plants, including oil palm and rice, showed a comparable stomatal density with C4 plants, including sugarcane and sorghum (Figures 1A, B), suggesting the similar stomatal pattern and production of monocots. However, rice showed a much lower stomatal index compared to the others due to its relatively higher number of pavement cells (Figures 1B, C). After drought treatment, the stomatal density and index of C3 plants, including Arabidopsis, oil palm and rice, were reduced, showing that drought represses the stomatal development of C3 plants. However, the stomatal development of C4 plants including sugarcane and sorghum were not affected by drought stress (Figure 1B). The stomatal index was measured to determine whether the possible reduction of the total epidermal cells caused the reduction in stomatal density. All the stomatal indexes of C3 and C4 plant groups showed the same trend with their stomatal densities after drought treatment (Figures 1B, C). The stomatal indexes of C3 plants were reduced whereas those of C4 plants were unchanged (Figure 1C), indicating that drought repressed the stomatal development of C3 plants but not C4 plants.
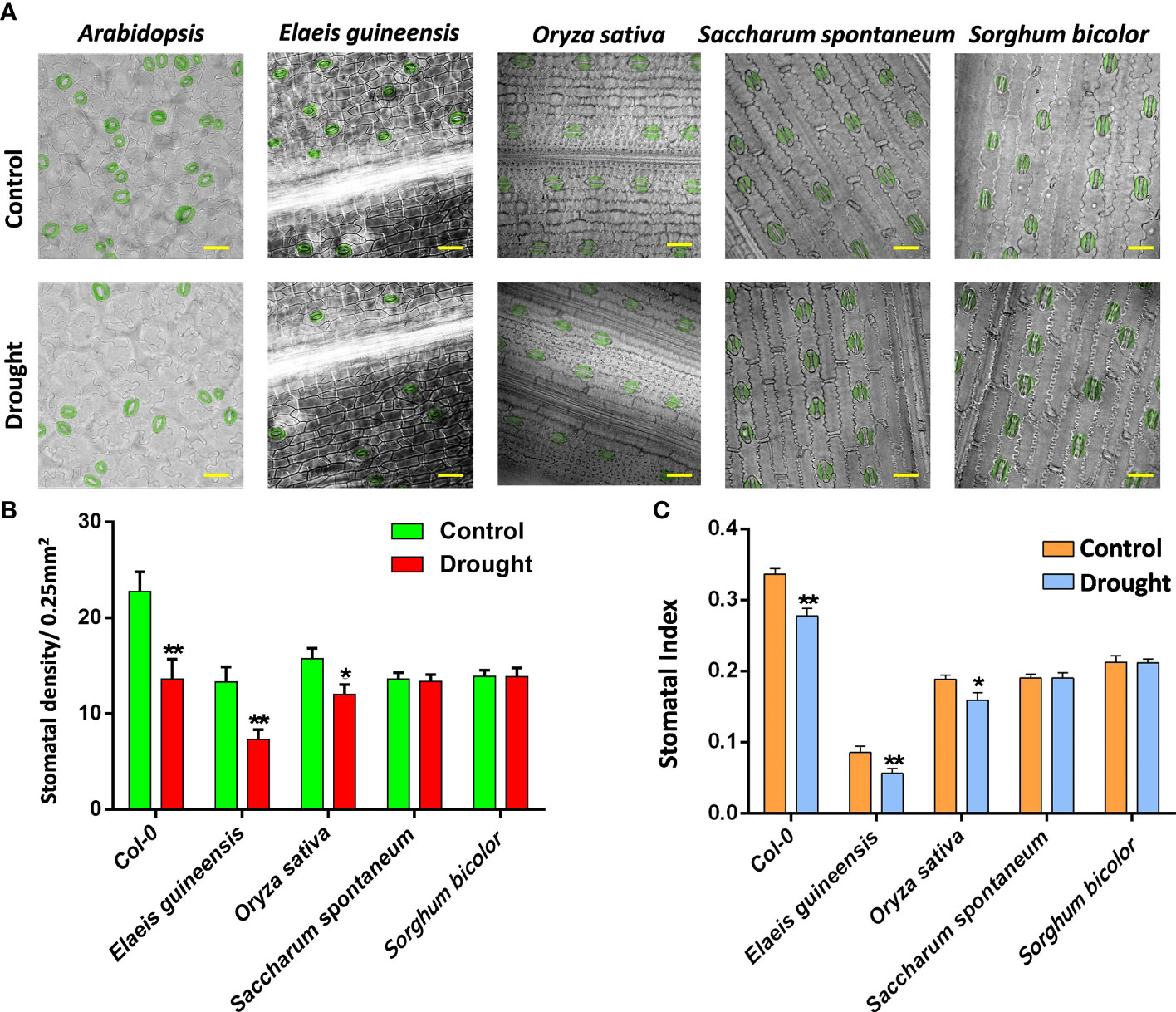
Figure 1 (A) The abaxial stomatal pattern of 14 dpg Arabidopsis, two-year-old Elaeis guineesis, 21 dpg Oryza sativa, one-year-old Saccharum spontaneum and one-year-old Sorghum bicolor under control and drought stress. Bar =25 um. (B) The stomatal density of samples from (A). (C) The stomatal index of samples from (A). The values are mean ± SEM; n ≥ 20. One-way ANOVA with post hoc Tukey HSD; *, p <0.05; **, p<0.01.
Stomatal response to drought stress in drought-tolerant oil palm and sugarcane
The stomatal response to drought stress of drought-tolerant C3 and C4 plants, compared to their drought-susceptible control, was tested using oil palm and sugarcane (Figures 2A, D). After drought treatment, leaf yellowing, leaf top necrosis, and other morphological changes were observed in both the drought-susceptible oil palm and sugarcane, although sugarcane could endure longer periods without water before its growth was affected (Figures 2A, D). However, the growth of drought-tolerant C3 and C4 crops was not affected (Figures 2A, D). The stomatal development of drought-tolerant oil palm and drought-susceptible sugarcane was unresponsive to drought stress (Figures 2B, C, E, F), which showed that the drought-susceptible sugarcane naturally has a higher tolerance to drought compared to C3 crops. Interestingly, the drought-tolerant sugarcane showed reduced stomatal development similar to a drought-susceptible C3 crop. (Figures 2E, F). In addition, drought-tolerant oil palm showed a lower stomatal density compared to the drought-susceptible oil palm (Figures 2B, C) whereas the stomatal density of drought-tolerant sugarcane was higher than the drought-susceptible sugarcane. After a 21 dpg (Figure 2D) intense drought treatment, the stomatal density was reduced to the same level of the susceptible sugarcane (Figures 2E, F). These data suggest the different physiological responses and molecular mechanisms of C3 and C4 crops under drought stress.
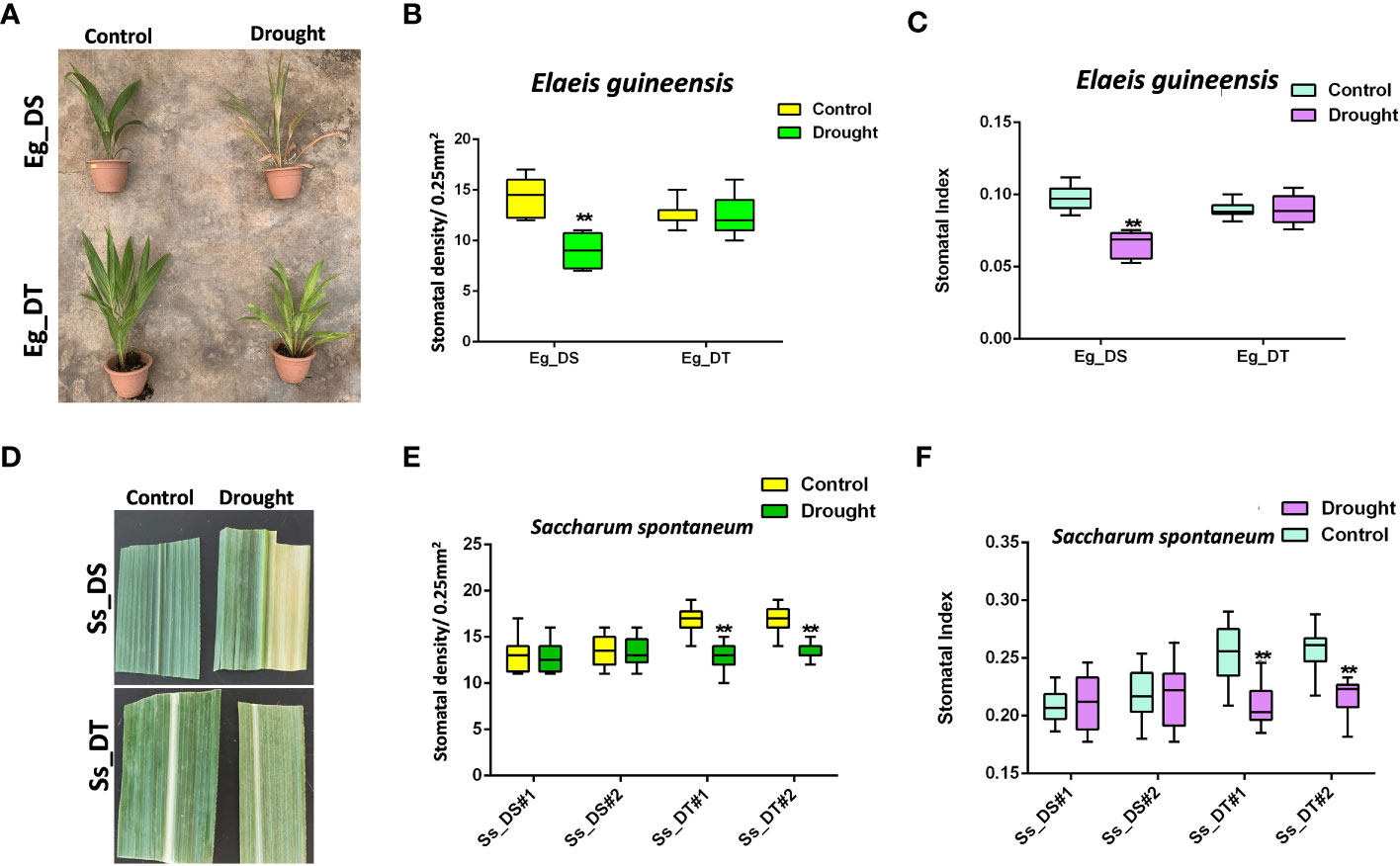
Figure 2 (A) The drought assay of drought-susceptible (Eg_DS) and drought-tolerant (Eg_DT) oil palms. Two-year-old oil palms were either continually watered or not watered for 14 days; n ≥ 4. (B) The stomatal density of samples from (A). (C) The stomatal index of samples from (A). (D) The drought assay of drought-susceptible (Ss_DS) and drought-tolerant (Ss_DT) sugarcanes. (E) The stomatal density of samples from (D). (F) The stomatal index of samples from (D). One-year-old sugarcanes were either continually watered or not watered for 21 days; n ≥ 2. The values are mean ± SEM; n ≥ 20. One-way ANOVA with post hoc Tukey HSD; **, p<0.01.
Genetic divergence of stomatal regulators SPCH, MUTE and FAMA in C3 and C4 plants
In general, genetic divergence of SPCH, MUTE and FAMA was found between C3 and C4 plants (Figure 3). Only a few C3 crops had homologs of the above three proteins (Figure 3). In contrast, there were 2–3 groups of homologs for each of SPCH, MUTE, and FAMA in C4 plants (Figure 3 and Supplemental Table S1). Each group contained several homologs with highly conserved genetic similarity. The homologs were on either homologous or different chromosomes (Figure 3 and Supplemental Table S1). The MUTE and FAMA proteins of C3 and C4 plants were highly differentiated (Figure 3). However, C4 plants exhibited two genetic patterns of MUTE and FAMA (Figure 3). SsFT2 and ZmFT6 showed typical C3-like pattern, SsSPCH2-1/2-2, SbSPCH2 and ZmSPCH2 were closer to the C3 SPCH group (Figure 3). Interestingly, among the C3 plants used for our phytogenic analysis, rice showed the closest genetic relationship with C4 plants (Figure 3). The FT and SPCH of rice showed an intermediate genetic pattern between not only C3 and C4, but also a C3-like C4 group and a C4-pattern C4 group (Figure 3). These data mirrored the different pace of evolution of SPCH and FT proteins from C3 to C4 plants.
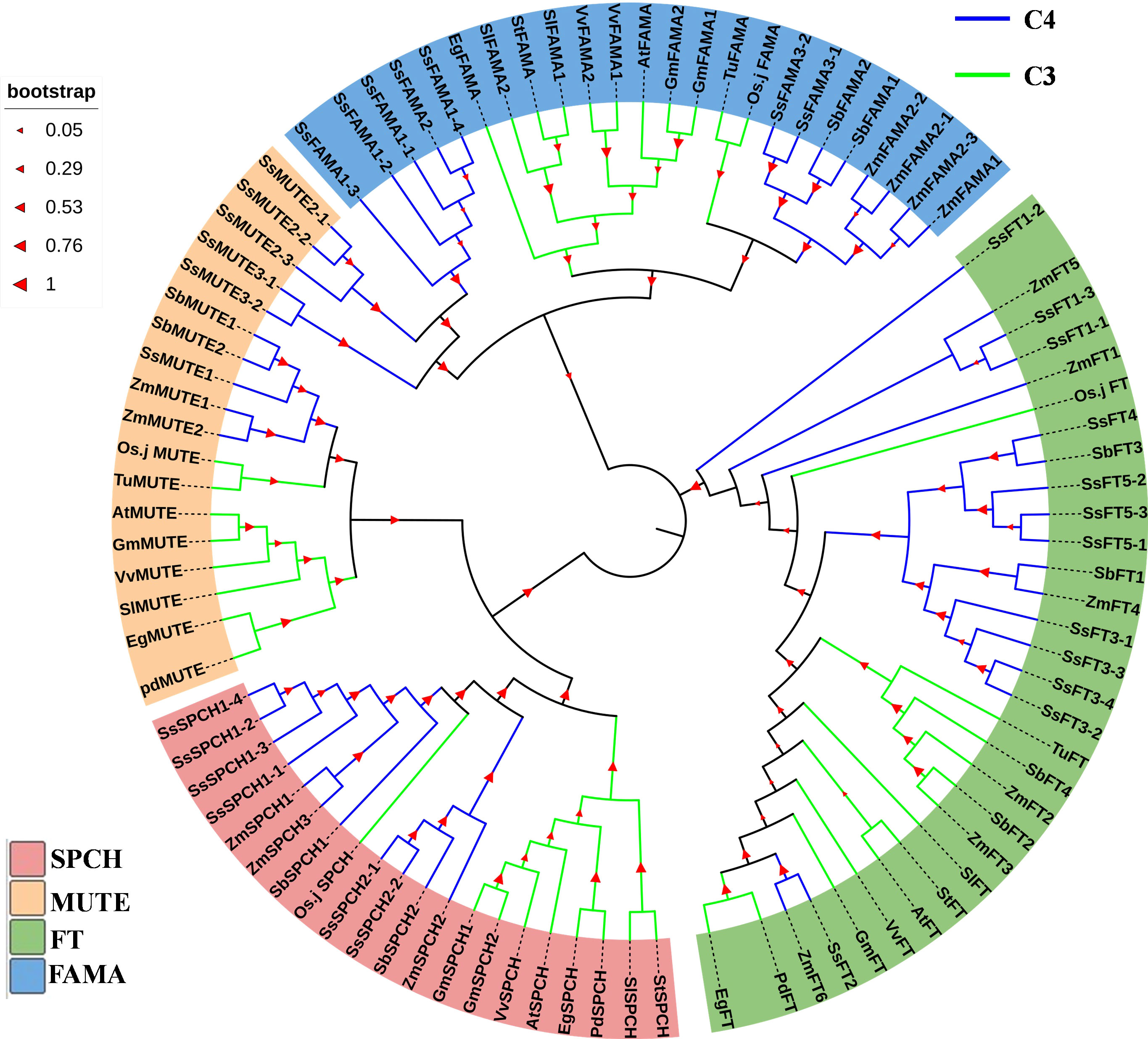
Figure 3 The phylogenic analysis of the protein of SPCH and its two homolog transcription factors MUTE and FAMA in Arabidopsis (At), Elaeis guineensis (Eg), Phoenix dactylifera (Pd), Oryza Sativa.japonica (Osj), Gycine max (Gm), Triticum urartu (Tu), Solanum lycopersicum (Sl), Solanum tuberosum (St), Vitis vinifera (Vv), Zea mays (Zm), Sorghum bicolor (Sb), Saccharum spontaneum (Ss). The protein FLOWERING LOCUS T (FT) of above species was used as the outgroup. Bootstrap =500; The percentage of replicate trees in which the associated taxa clustered together in the bootstrap test (500 replicates) is shown on the branches.
Divergent expression of SPCH in response to drought stress
The expressions of SPCH, MUTE, and FAMA in oil palm and rice were downregulated in the drought-susceptible plants under drought stress (Figures 4C, D). However, the expressions of these three genes were basically unchanged in the drought-tolerant oil palm under drought stress (Figure 4C), suggesting the importance of the SPCH signalling pathway in drought tolerance.
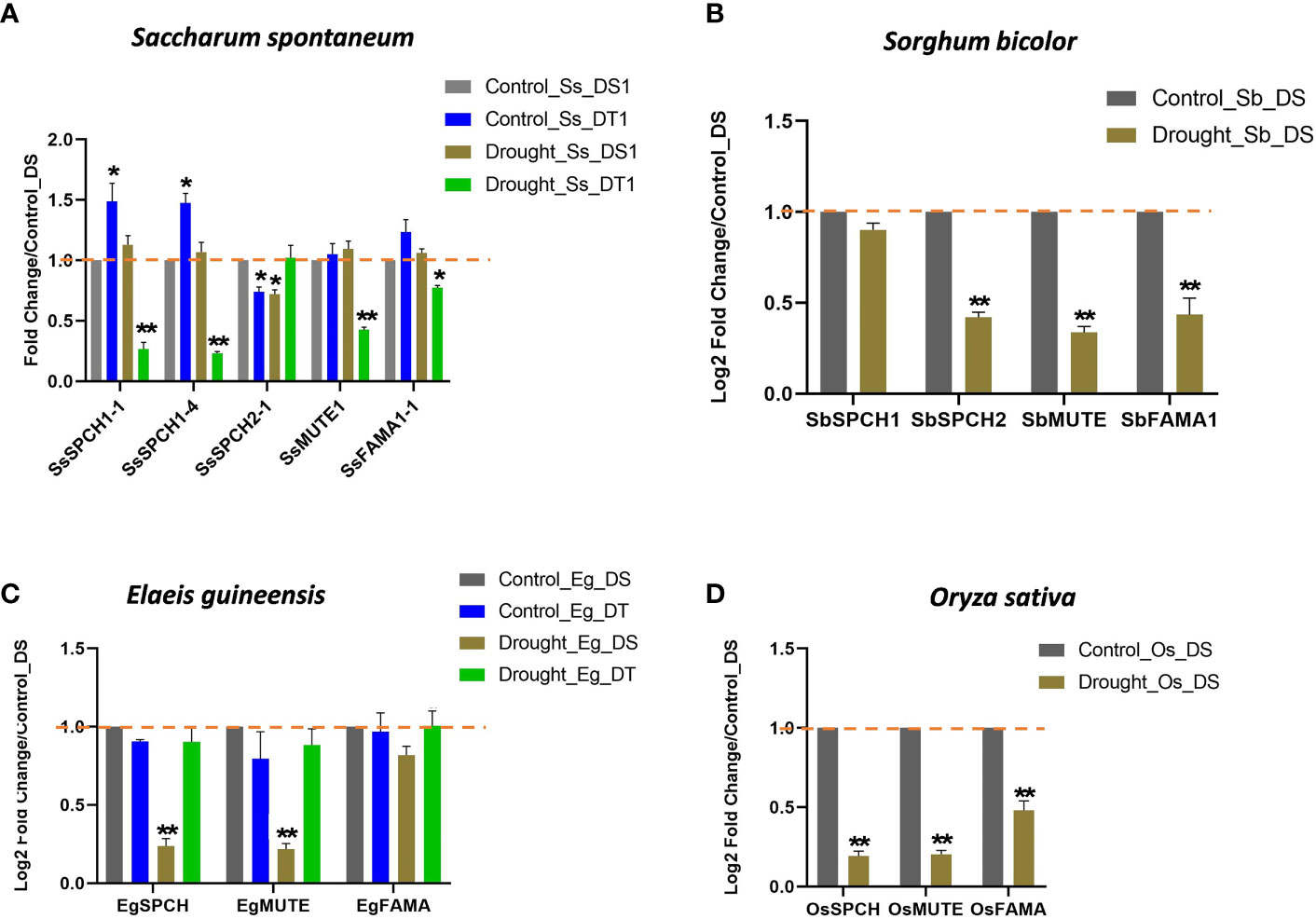
Figure 4 The relative expression levels of SPCH, MUTE and FAMA in (A) the drought-susceptible and tolerant Saccharum spontaneum; (B) Sorghum bicolor; (C) the drought-susceptible and tolerant Elaeis guineensis and (D) Oryza sativa. Triplicates were included in the Q-PCR, the expression level of drought-susceptible plants under control was standardized to 1. The values are mean ± SEM; n ≥ 3. One-way ANOVA with post hoc Tukey HSD; *, p <0.05; **, p<0.01.
The expressions of three sugarcane SPCH-SsSPCH1-1, SsSPCH1-4, SsSPCH2-1 (C3-like) and two sorghum SPCH- SbSPCH1, SbSPCH2 (C3-like) were analysed (Figures 4A, B). Interestingly, C4 and C3-like SPCH showed different expressions in response to drought (Figures 4A, B). Drought stress had few effects on the expression of C4 SPCH including SsSPCH1-1, SsSPCH1-4 and SbSPCH1 in drought-susceptible sugarcane and sorghum but repressed the expressions of C3-like SsSPCH1-3 and SbSPCH2 (Figures 4A, B). By contrast, drought strongly repressed the expressions of SsSPCH1 and SsSPCH2 but not SsSPCH1-3 in drought-tolerant sugarcane (Figures 4A, B). Taken together, these data and the stomatal response to drought suggest that the regulation of C4 SPCH plays a dominant role in regulating the stomatal response of C4 plants to drought stress
The structural difference of SPCH in C3 and C4 plants
The protein sequence and 3D protein structure of the C3 and C4 SPCH were analysed to determine the structural divergence of proteins, and their possible association with the phosphorylation of SPCH (Figure 5). Although SPCH of Arabidopsis and sugarcane showed a highly conserved helix-loop-helix (bHLH) domain (Supplemental Figure S1) and a similar bHLH structure (Figures 5A, B, blue), the folding of the peptide and structures of other domains were largely different (Figures 5A, B). Specifically, the MAPK target domain (MPKTD) of C3 and C4 SPCH exhibited high diversity (Figures 5C, D), suggesting the different phosphorylation sensitivity of C3 and C4 SPCH to upstream MAPKs. In summary, 17 C3-specific and 4 C4-specific Serine/Threonine (S/T) phosphorylation sites were found within the MPKTD (Figure 5C). Interestingly, 18 C3-like C4-specific S/Ts were found (Figure 5C), suggesting the SPCH homologs in C3 and C4 plants may exhibit different expressions at both transcriptional and post-translational levels. C3- and C4- specific insert/deletion (InDel) polymorphisms were found in the MPKTD, which may also affect the function of SPCH. In addition, the upstream (N-Terminus) of SPCH in C3 and C4 crops also showed high polymorphism, including SNPs and InDels (Supplemental Figure S1). These data indicate that the functional domain and sequences of C3 and C4 SPCH protein have undergone different evolution, which may lead to different levels of phosphorylation via MPKs signalling pathways and the stomatal lineage cell transition
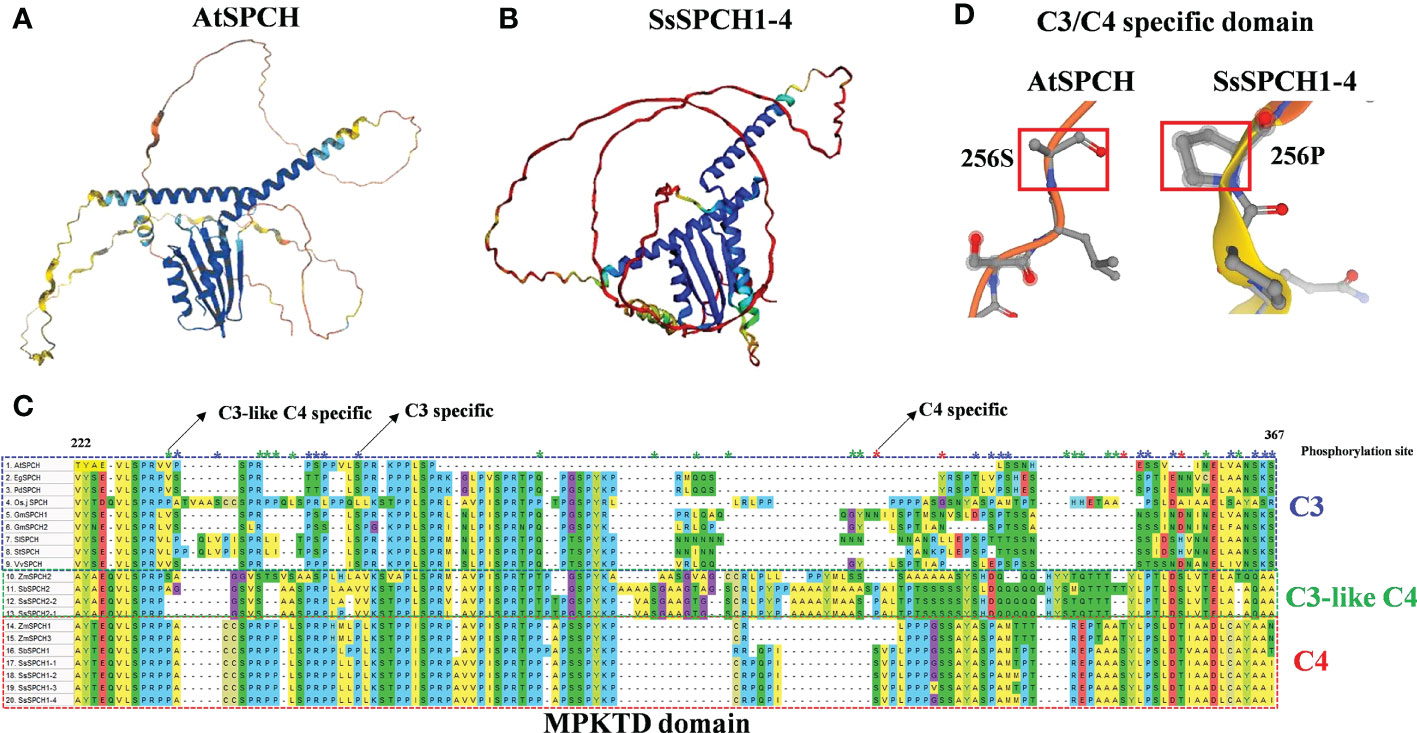
Figure 5 The 3D protein structure of (A) Arabidopsis SPCH and (B) Sugarcane SPCH1-4; (C) The alignment of MPKTD domains of C3 and C4 plants. The blue, red and green frame indicate the MPKTD sequence of C3, C4 and C3-like C4 SPCH. The blue, red and green asterisks indicate the C3-, C4, and C3-like C4- specific phosphorylation sites (S/T) within the MPKTD domain; (D) The C3/C4 specific S/T site (256) of AtSPCH and SssSPCH1-4.
Discussion
Stomatal development of C3 and C4 plants in response to drought stress
Crops exhibit different drought tolerances. To ensure that each species underwent adequate drought stress, the plants were treated with different durations of drought according to previous studies (Aharoni et al., 2004; Hura et al., 2007; Quan et al., 2010; Jangpromma et al., 2012; Zhang et al., 2015; Wang et al., 2020). Arabidopsis and rice seedlings show lower drought tolerance, therefore they were not given water for seven days (Aharoni et al., 2004; Quan et al., 2010). Tropical C3 crops like oil palm show a relatively higher drought tolerance than other temperate climate-grown C3 crops, thus, a 14-day drought treatment was used for oil palm as previously described (Wang et al., 2020). C4 plants were naturally more tolerant to drought than C3 plants (Hura et al., 2007). Seven- to nine-day drought assays were used in previous studies of sugarcane (Jangpromma et al., 2012; Zhang et al., 2015). However, a 9-day drought treatment did not induce obvious growth repression in our sugarcane (data not shown). Thus, we extended the drought treatment to 14 days until leaf yellowing was found in some sugarcane.
The result showed that drought strongly repressed the stomatal development of C3 plants but did not affect that of C4 plants (Figure 1), suggesting that C4 plants exhibit higher drought tolerance at the cellular level. The stabilization of stomatal development under drought may be helpful for C4 plants to maintain their photosynthesis efficiency. In addition, as sibling species, sugarcane and sorghum exhibited almost the same stomatal developmental pattern and response to drought (Figure 1). By contrast, the stomatal developmental pattern varied among C3 plants (Figure 1).
We obtained the drought-tolerant seedlings of two tropical crops, oil palm (Wang et al., 2020) and sugarcane, via drought assays. The data revealed that the stomatal development of drought-tolerant oil palm was not affected by drought (Figures 2B, C), which was similar to drought-susceptible sugarcane (Figures 2E, F). This result is consistent with the results in other drought-tolerant C3 crops where the cell development and homeostasis of physiological processes are not affected by drought stress (Mehri et al., 2009; Yoo et al., 2010; Liu et al., 2012). Therefore, the maintenance of stomatal development is required for stabilizing the biological process of C3 plants during drought.
Although C4 plants show a relatively higher drought tolerance compared to C3 plants (Taylor et al., 2010), long periods of drought still affected the growth and development of sugarcane (Figure 2D). Nevertheless, the stomatal development was not affected by drought stress (Figures 2E, F). Interestingly, the stomatal development of drought-tolerant sugarcane was repressed by drought (Figures 2E, F). These data suggest that during the evolution of C4 plants, the stomatal developmental system may have evolved to be unresponsive to drought stress, which would facilitate gas exchange during highly efficient photosynthesis. However, only drought-tolerant C4 plants may further protect themselves from drought damage by reducing water through ‘sensing’ the drought signal via their stomata. To validate this hypothesis, it would be interesting to test the stomatal response to drought in more C4 species.
SPCH homologs undergoes differential evolution in C4 plants
Although the sugarcane gnomes have been sequenced at the monoploid (Garsmeur et al., 2018) and polyploid levels (Zhang et al., 2018), the extreme complexity of the sugarcane genome makes it difficult to analyze and annotate the genome (Thirugnanasambandam et al., 2018). The modern sugarcane has a duplicated genome originating from S. officinarum and S. spontaneum (D’hont et al., 2008). We identified at least six SPCH homologs in the polyploid genome (Zhang et al., 2018) to investigate the effects of gene duplication on the function of stomatal regulators. Our result showed that MUTE and FAMA were specifically evolved between C3 and C4 plants (Figure 3). In contrast, SPCH homologs of C4 species exhibited C4 and C3-like patterns (Figure 3). These data indicate the divergent evolution of SPCH homologs in C4 crops. The varied chromosomal structure, the interspecific hybridization, and the diverse growth habitat may play a key role in the duplication and evolution of sugarcane homologs (Thirugnanasambandam et al., 2018). As a key regulator of the rapid response of stomata to the environment (Lau et al., 2018), sugarcane SPCH homologs may have originated from ancestors that underwent divergent selection (Thirugnanasambandam et al., 2018). However, the SPCH homologs of C4 plants in this study might not cover all the homologs due to the complexity of the C4 plant genomes. Improved genome assemblies of C4 crops in the future would be helpful to better understand the functional genetics of sugarcane in response to environmental stresses.
In our study, the genetic relationship of rice’s FT and SPCH are in between of the C4-type and C3-like FT & SPCH of C4 plants (Figure 3). Our study supports the previous study that some rice genes have rapidly evolved into C4-like pattern (Wang et al., 2009). These studies pave the way to bio-engineer C4 rice (Ermakova et al., 2020).
Regulation of SPCH expression at transcriptional and post-translational level
As a master transcription factor, the transcription of SPCH is also regulated by other transcription factors under different environmental changes (Lau et al., 2014; Lau et al., 2018). Interestingly, the SPCH homologs showed different expressional changes in response to drought stress (Figures 4A, B). Expressions of SsSPCH1-1, SsSPCH1-4 and SbSPCH1 were unresponsive to drought in drought-susceptible C4 plants but were repressed in drought-tolerant C4 plants (Figures 4A, B), which explained their cellular response to drought stress (Figure 2). The pseudogenes are largely identified in the sugarcane genome (Monteiro-Vitorello et al., 2004). In our study, SsSPCH2-1 and SbSPCH2 showed a C3-like expression repressed by drought stress (Figures 4A, B), which was opposite to the stomatal response of C4 plants to drought (Figures 2D-F). It was hypothesized that the C3-like SPCH in C4 plants may either be non-functional pseudogenes or have other drought-independent functions. Further functional validations of the promoter activities of these homolog genes would be helpful to test which of these homologs are functional and which of them are pseudogenes. Despite the difficulty in testing the function of each SPCH gene in C4 plants, our research reveals the transcriptional response of key stomatal regulators in C4 crops under drought stress. The divergent expressions of C4 SPCH homologs are associated with the drought response of C4 plants at the cellular level.
In C3 plants, drought also represses SPCH expression at the protein level via the MPK3/6 signaling pathway (Lee and Bergmann, 2019) and other kinase-dependent pathways (Yang et al., 2022). Serine/Threonine residues are required for the binding of MAPKs and SPCH, resulting in the phosphorylation of SPCH (Lampard et al., 2008). Although the bHLH domains of SPCH were highly conserved in plants (Supplemental Figure S1), the folding structure and key MPKTD domains were largely different (Figure 5), which might lead to different binding affinity of SPCH to both upstream kinases and downstream target genes, resulting in the different expression of SPCH at post-translational level in response to drought stress. The bHLH domain is required for the brassinosteroid dependent stomatal formation (De Marcos et al., 2017). There are S/T phosphorylation sites located upstream of SPCH which are also required for its function in stomatal lineage cell transition (Davies and Bergmann, 2014). Although the bHLH domain is highly conserved across C3 and C4 plants, the N-terminus, C-terminus and other functional domains of C3 and C4 SPCH showed high polymorphism (Figure 5) indicating the possible functional diversity of the SPCH homologs in initiating stomatal lineage cell development.
Crosslinks of gene expression, stomatal development and drought tolerance
The relationship between gene regulatory networks of stomatal plasticity and osmotic stresses has been largely studied in C3 model plants and crops. For example, the alternative expression of SPCH via transcriptional and post-translational regulation directly affects stomatal production (Lampard et al., 2008; Lau et al., 2014; Lau et al., 2018), resulting in a change in drought tolerance (Hepworth et al., 2015; Yang et al., 2022). In this study, we scaled up the investigation of this relationship to C3 crops grown in tropical areas where drought stress is a big challenge for food security (Oliveira et al., 2021). We validated that the existing gene regulatory networks also worked in tropical crops (Figures 2A–C, 4C). Importantly, the crosslinks of SPCH expression, stomatal production, and drought response were different in C4 crops (Figure 6). The homologs of SPCH in C4 plants have undergone a complex evolution (Figure 3) and showed divergent expression responses to drought stress (Figures 4A, B).
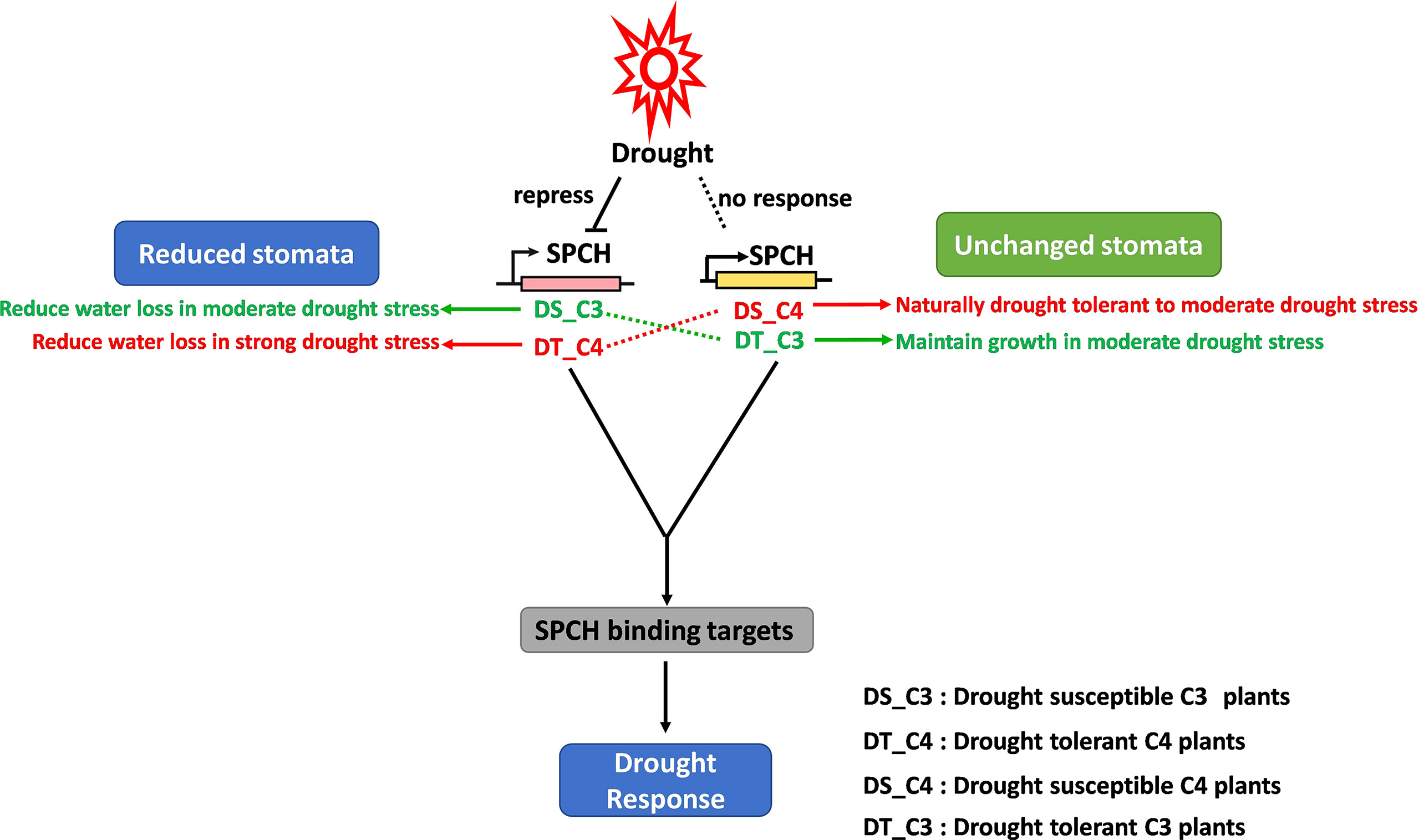
Figure 6 The proposed stomatal response to drought stress regulated by the divergent expression of SPCH in C3 and C4 plants. Drought repressed the expression of the SPCH of C3 plants but had no effect on that of C4 plants, resulting in the reduction of stomata in C3 plants, and no change in stomata in C4 plants in response to drought stress. However, drought-tolerant C3 plants showed no stomatal response to drought due to the stabilized expression of SPCH under drought stress. Conversely, drought-tolerant C4 plants were able to reduce the production of stomata and limit the water loss due to the re-activation of SPCH response to drought.
In conclusion, we identified the molecular difference of C3 and C4 SPCH at transcriptional level and protein level. We proposed a working model including the drought response of both drought-susceptible and drought-tolerant C3 and C4 crops (Figure 6). Our model supports the studies in C3 plants that drought represses the stomatal development via inhibiting the SPCH expression whereas the stomatal development and SPCH expression level are not affected in drought-tolerant C3 crops (Aharoni et al., 2004; Quan et al., 2010). In contrast, the expression of C4 SPCH was not affected by drought stress, which might lead to the stabilization of stomatal development of C4 plants during drought stress (Figure 6). However, drought-tolerant sugarcane exhibited C3 plant-like response of SPCH expression and stomatal development (Figure 6), suggesting the different upstream regulation of SPCH expression in drought-tolerant and drought-susceptible C4 plants. According to this result, the drought-tolerant sugarcane was able to reduce water loss via regulation of stomatal development to further increase the tolerance to drought stress. It would be interesting to investigate the underlying genomic and epigenetic mechanism of how SPCH is differentially regulated in drought-susceptible and tolerant crops. This research is also helpful in understanding the evolution of the key functional genes and their roles in drought tolerance.
Data availability statement
The original contributions presented in the study are included in the article/Supplementary Materials, further inquiries can be directed to the corresponding author/s.
Author contributions
GY conceived and supervised the project. ZS designed the study. ZS, LW and ML conducted the experiments. ZS and LW analyzed the data. ZS and GY prepared the manuscript. All authors contributed to the article and approved the submitted version.
Funding
This work was supported by the Internal Funds of the Temasek Life Sciences Laboratory.
Acknowledgments
We thank our lab members for critical comments and taking care of the plants.
Conflict of interest
The authors declare that the research was conducted in the absence of any commercial or financial relationships that could be construed as a potential conflict of interest.
Publisher’s note
All claims expressed in this article are solely those of the authors and do not necessarily represent those of their affiliated organizations, or those of the publisher, the editors and the reviewers. Any product that may be evaluated in this article, or claim that may be made by its manufacturer, is not guaranteed or endorsed by the publisher.
Supplementary material
The Supplementary Material for this article can be found online at: https://www.frontiersin.org/articles/10.3389/fpls.2023.1100838/full#supplementary-material
References
Aharoni, A., Dixit, S., Jetter, R., Thoenes, E., Van Arkel, G., Pereira, A. (2004). The SHINE clade of AP2 domain transcription factors activates wax biosynthesis, alters cuticle properties, and confers drought tolerance when overexpressed in arabidopsis. Plant Cell 16, 2463–2480. doi: 10.1105/tpc.104.022897
Basu, S., Ramegowda, V., Kumar, A., Pereira, A. (2016). Plant adaptation to drought stress. F1000 5, 1554. doi: 10.12688/f1000research.7678.1
Chater, C. C., Oliver, J., Casson, S., Gray, J. E. (2014). Putting the brakes on: Abscisic acid as a central environmental regulator of stomatal development. New Phytol. 202, 376–391. doi: 10.1111/nph.12713
Davies, K. A., Bergmann, D. C. (2014). Functional specialization of stomatal bHLHs through modification of DNA-binding and phosphoregulation potential. Proc. Natl. Acad. Sci. U.S.A. 111, 15585–15590. doi: 10.1073/pnas.1411766111
De Marcos, A., Houbaert, A., Triviño, M., Delgado, D., Martín-Trillo, M., Russinova, E., et al. (2017). A mutation in the bHLH domain of the SPCH transcription factor uncovers a BR-dependent mechanism for stomatal development. Plant Physiol. 174, 823–842. doi: 10.1104/pp.17.00615
D’hont, A., Souza, G. M., Menossi, M., Vincentz, M., Van-Sluys, M.-A., Glaszmann, J. C., et al. (2008). Sugarcane: A major source of sweetness, alcohol, and bio-energy. Genomics Trop. Crop Plants, 1:483–513. doi: 10.1007/978-0-387-71219-2_21
Dinneny, J. R. (2019). Developmental responses to water and salinity in root systems. Annu. Rev. Cell Dev. Biol. 35, 239–257. doi: 10.1146/annurev-cellbio-100617-062949
Ermakova, M., Danila, F. R., Furbank, R. T., Von Caemmerer, S. (2020). On the road to C4 rice: advances and perspectives. Plant J. 101, 940–950. doi: 10.1111/tpj.14562
Garsmeur, O., Droc, G., Antonise, R., Grimwood, J., Potier, B., Aitken, K., et al. (2018). A mosaic monoploid reference sequence for the highly complex genome of sugarcane. Nat. Commun. 9, 2638. doi: 10.1038/s41467-018-05051-5
Gupta, A., Rico-Medina, A., Caño-Delgado, A. I. (2020). The physiology of plant responses to drought. Science 368, 266–269. doi: 10.1126/science.aaz7614
Han, S.-K., Kwak, J. M., Qi, X. (2021). Stomatal lineage control by developmental program and environmental cues. Front. Plant Sci., 2193. doi: 10.3389/fpls.2021.751852
Hepworth, C., Doheny-Adams, T., Hunt, L., Cameron, D. D., Gray, J. E. (2015). Manipulating stomatal density enhances drought tolerance without deleterious effect on nutrient uptake. New Phytol. 208, 336–341. doi: 10.1111/nph.13598
Hung, J.-H., Weng, Z. (2016). Sequence alignment and homology search with BLAST and ClustalW. Cold Spring Harb. Protoc. 2016, prot093088. doi: 10.1101/pdb.prot093088
Hura, T., Hura, K., Grzesiak, M., Rzepka, A. (2007). Effect of long-term drought stress on leaf gas exchange and fluorescence parameters in C3 and C4 plants. Acta Physiol. Plant 29, 103–113. doi: 10.1007/s11738-006-0013-2
Hussain, Q., Asim, M., Zhang, R., Khan, R., Farooq, S., Wu, J. (2021). Transcription factors interact with ABA through gene expression and signaling pathways to mitigate drought and salinity stress. Biomolecules 11, 1159. doi: 10.3390/biom11081159
Jangpromma, N., Thammasirirak, S., Jaisil, P., Songsri, P. (2012). Effects of drought and recovery from drought stress on above ground and root growth, and water use efficiency in sugarcane (Saccharum officinarum l.). Aust. J. Crop Sci. 6, 1298–1304.
Jumper, J., Evans, R., Pritzel, A., Green, T., Figurnov, M., Ronneberger, O., et al. (2021). Highly accurate protein structure prediction with AlphaFold. Nature 596, 583–589. doi: 10.1038/s41586-021-03819-2
Kanaoka, M. M., Pillitteri, L. J., Fujii, H., Yoshida, Y., Bogenschutz, N. L., Takabayashi, J., et al. (2008). SCREAM/ICE1 and SCREAM2 specify three cell-state transitional steps leading to Arabidopsis stomatal differentiation. Plant Cell 20, 1775–1785. doi: 10.1105/tpc.108.060848
Kumari, A., Jewaria, P. K., Bergmann, D. C., Kakimoto, T. (2014). Arabidopsis reduces growth under osmotic stress by decreasing SPEECHLESS protein. Plant Cell Physiol. 55, 2037–2046. doi: 10.1093/pcp/pcu159
Kumar, S., Stecher, G., Li, M., Knyaz, C., Tamura, K. (2018). MEGA X: Molecular evolutionary genetics analysis across computing platforms. Mol. Biol. Evol. 35, 1547–1549. doi: 10.1093/molbev/msy096
Lampard, G. R., Macalister, C. A., Bergmann, D. C. (2008). Arabidopsis stomatal initiation is controlled by MAPK-mediated regulation of the bHLH SPEECHLESS. Science 322, 1113–1116. doi: 10.1126/science.1162263
Lau, O. S., Davies, K. A., Chang, J., Adrian, J., Rowe, M. H., Ballenger, C. E., et al. (2014). Direct roles of SPEECHLESS in the specification of stomatal self-renewing cells. Science 345, 1605–1609. doi: 10.1126/science.1256888
Lau, O. S., Song, Z., Zhou, Z., Davies, K. A., Chang, J., Yang, X., et al. (2018). Direct control of SPEECHLESS by PIF4 in the high-temperature response of stomatal development. Curr. Biol. 28, 1273–1280. e3. doi: 10.1016/j.cub.2018.02.054
Lee, L. R., Bergmann, D. C. (2019). The plant stomatal lineage at a glance. J. Cell Sci. 132, jcs228551. doi: 10.1242/jcs.228551
Liu, X., Liu, S., Zhang, J., Wu, Y., Wu, W., Zhang, Y., et al. (2020). Optimization of reference genes for qRT-PCR analysis of microRNA expression under abiotic stress conditions in sweetpotato. Plant Physiol. Biochem. 154, 379–386. doi: 10.1016/j.plaphy.2020.06.016
Liu, J., Zhang, F., Zhou, J., Chen, F., Wang, B., Xie, X. (2012). Phytochrome b control of total leaf area and stomatal density affects drought tolerance in rice. Plant Mol. Biol. 78, 289–300. doi: 10.1007/s11103-011-9860-3
Macalister, C. A., Ohashi-Ito, K., Bergmann, D. C. (2007). Transcription factor control of asymmetric cell divisions that establish the stomatal lineage. Nature 445, 537–540. doi: 10.1038/nature05491
Mcginnis, S., Madden, T. L. (2004). BLAST: At the core of a powerful and diverse set of sequence analysis tools. Nucleic Acids Res. 32, W20–W25. doi: 10.1093/nar/gkh435
Mehri, N., Fotovat, R., Saba, J., Jabbari, F. (2009). Variation of stomata dimensions and densities in tolerant and susceptible wheat cultivars under drought stress. J. Food Agric. Environ. 7, 167–170.
Mirdita, M., Schütze, K., Moriwaki, Y., Heo, L., Ovchinnikov, S., Steinegger, M. (2022). ColabFold: making protein folding accessible to all. Nat. Methods, 19:679–682. doi: 10.1038/s41592-022-01488-1
Monteiro-Vitorello, C. B., Camargo, L. E., Van Sluys, M. A., Kitajima, J. P., Truffi, D., Do Amaral, A. M., et al. (2004). The genome sequence of the gram-positive sugarcane pathogen Leifsonia xyli subsp. xyli. Mol. Plant Microbe Interact. 17, 827–836. doi: 10.1094/MPMI.2004.17.8.827
Ogura, T., Goeschl, C., Filiault, D., Mirea, M., Slovak, R., Wolhrab, B., et al. (2019). Root system depth in arabidopsis is shaped by EXOCYST70A3 via the dynamic modulation of auxin transport. Cell 178, 400–412. e16. doi: 10.1016/j.cell.2019.06.021
Ohashi-Ito, K., Bergmann, D. C. (2006). Arabidopsis FAMA controls the final proliferation/differentiation switch during stomatal development. Plant Cell 18, 2493–2505. doi: 10.1105/tpc.106.046136
Oh, J. E., Kwon, Y., Kim, J. H., Noh, H., Hong, S.-W., Lee, H. (2011). A dual role for MYB60 in stomatal regulation and root growth of Arabidopsis thaliana under drought stress. Plant Mol. Biol. 77, 91–103. doi: 10.1007/s11103-011-9796-7
Oliveira, R. S., Eller, C. B., Barros, F. D. V., Hirota, M., Brum, M., Bittencourt, P. (2021). Linking plant hydraulics and the fast–slow continuum to understand resilience to drought in tropical ecosystems. New Phytol. 230, 904–923. doi: 10.1111/nph.17266
Pillitteri, L. J., Sloan, D. B., Bogenschutz, N. L., Torii, K. U. (2007). Termination of asymmetric cell division and differentiation of stomata. Nature 445, 501–505. doi: 10.1038/nature05467
Qi, J., Song, C. P., Wang, B., Zhou, J., Kangasjärvi, J., Zhu, J. K., et al. (2018). Reactive oxygen species signaling and stomatal movement in plant responses to drought stress and pathogen attack. J. Integr. Plant Biol. 60, 805–826. doi: 10.1111/jipb.12654
Quan, R., Hu, S., Zhang, Z., Zhang, H., Zhang, Z., Huang, R. (2010). Overexpression of an ERF transcription factor TSRF1 improves rice drought tolerance. Plant Biotechnol. J. 8, 476–488. doi: 10.1111/j.1467-7652.2009.00492.x
Sage, R. F. (2004). The evolution of C4 photosynthesis. New Phytol. 161, 341–370. doi: 10.1111/j.1469-8137.2004.00974.x
Sharwood, R. E., Ghannoum, O., Whitney, S. M. (2016). Prospects for improving CO2 fixation in C3-crops through understanding C4-rubisco biogenesis and catalytic diversity. Curr. Opin. Plant Biol. 31, 135–142. doi: 10.1016/j.pbi.2016.04.002
Song, L., Huang, S.-S. C., Wise, A., Castanon, R., Nery, J. R., Chen, H., et al. (2016). A transcription factor hierarchy defines an environmental stress response network. Science 354, aag1550. doi: 10.1126/science.aag1550
Song, Z., Wang, L., Lai, C., Lee, M., Yang, Z., Yue, G. (2022). EgSPEECHLESS responses to salt stress by regulating stomatal development in oil palm. Int. J. Mol. Sci. 23, 4659. doi: 10.3390/ijms23094659
Still, C. J., Berry, J. A., Collatz, G. J., Defries, R. S. (2003). Global distribution of C3 and C4 vegetation: carbon cycle implications. Glob. Biogeochem. Cycles. 17, 1029. doi: 10.1029/2001GB001807
Strimmer, K., Von Haeseler, A. (1996). Quartet puzzling: A quartet maximum-likelihood method for reconstructing tree topologies. Mol. Biol. Evol. 13, 964–969. doi: 10.1093/oxfordjournals.molbev.a025664
Taylor, S. H., Hulme, S. P., Rees, M., Ripley, B. S., Ian Woodward, F., Osborne, C. P. (2010). Ecophysiological traits in C3 and C4 grasses: a phylogenetically controlled screening experiment. New Phytol. 185, 780–791. doi: 10.1111/j.1469-8137.2009.03102.x
Thirugnanasambandam, P. P., Hoang, N. V., Henry, R. J. (2018). The challenge of analyzing the sugarcane genome. Front. Plant Sci. 9, 616. doi: 10.3389/fpls.2018.00616
Turck, F., Fornara, F., Coupland, G. (2008). Regulation and identity of florigen: FLOWERING LOCUS T moves center stage. Annu. Rev. Plant Biol. 59, 573–594. doi: 10.1146/annurev.arplant.59.032607.092755
Van Den Bergh, E., Külahoglu, C., Bräutigam, A., Hibberd, J. M., Weber, A. P., Zhu, X.-G., et al. (2014). Gene and genome duplications and the origin of C4 photosynthesis: birth of a trait in the Cleomaceae. Curr. Plant Biol. 1, 2–9. doi: 10.1016/j.cpb.2014.08.001
Visentin, I., Pagliarani, C., Deva, E., Caracci, A., Turečková, V., Novák, O., et al. (2020). A novel strigolactone-miR156 module controls stomatal behaviour during drought recovery. Plant Cell Environ. 43, 1613–1624. doi: 10.1111/pce.13758
Wang, X., Gowik, U., Tang, H., Bowers, J. E., Westhoff, P., Paterson, A. H. (2009). Comparative genomic analysis of C4 photosynthetic pathway evolution in grasses. Genome Biol. 10, R68. doi: 10.1186/gb-2009-10-6-r68
Wang, L., Lee, M., Ye, B., Yue, G. H. (2020). Genes, pathways and networks responding to drought stress in oil palm roots. Sci. Rep. 10, 21303. doi: 10.1038/s41598-020-78297-z
Yang, X., Gavya S, L., Zhou, Z., Urano, D., Lau, O. S. (2022). Abscisic acid regulates stomatal production by imprinting a SnRK2 kinase–mediated phosphocode on the master regulator SPEECHLESS. Sci. Adv. 8, eadd2063. doi: 10.1126/sciadv.add2063
Yoo, C. Y., Pence, H. E., Jin, J. B., Miura, K., Gosney, M. J., Hasegawa, P. M., et al. (2010). The arabidopsis GTL1 transcription factor regulates water use efficiency and drought tolerance by modulating stomatal density via transrepression of SDD1. Plant Cell 22, 4128–4141. doi: 10.1105/tpc.110.078691
Zhang, F.-J., Zhang, K.-K., Du, C.-Z., Li, J., Xing, Y.-X., Yang, L.-T., et al. (2015). Effect of drought stress on anatomical structure and chloroplast ultrastructure in leaves of sugarcane. Sugar Tech 17, 41–48. doi: 10.1007/s12355-014-0337-y
Keywords: drought, tolerance, C3, C4, stomata, SPEECHLESS
Citation: Song Z, Wang L, Lee M and Yue GH (2023) The evolution and expression of stomatal regulators in C3 and C4 crops: Implications on the divergent drought tolerance. Front. Plant Sci. 14:1100838. doi: 10.3389/fpls.2023.1100838
Received: 17 November 2022; Accepted: 02 January 2023;
Published: 01 February 2023.
Edited by:
Muhammad Ahsan Altaf, Hainan University, ChinaReviewed by:
Gothandapani Sellamuthu, Czech University of Life Sciences Prague, CzechiaGeetha Govind, University of Agricultural Sciences, Bangalore, India
Copyright © 2023 Song, Wang, Lee and Yue. This is an open-access article distributed under the terms of the Creative Commons Attribution License (CC BY). The use, distribution or reproduction in other forums is permitted, provided the original author(s) and the copyright owner(s) are credited and that the original publication in this journal is cited, in accordance with accepted academic practice. No use, distribution or reproduction is permitted which does not comply with these terms.
*Correspondence: Gen Hua Yue, Z2VuaHVhQHRsbC5vcmcuc2c=