- 1Zhengzhou Research Base, State Key Laboratory of Cotton Biology, School of Agricultural Sciences, Zhengzhou University, Zhengzhou, Henan, China
- 2State Key Laboratory of Cotton Biology, Institute of Cotton Research, Chinese Academy of Agricultural Sciences, Anyang, Henan, China
- 3College of Agronomy, Shenyang Agricultural University, Shenyang, China
- 4Engineering Research Centre of Cotton, Ministry of Education, Xinjiang Agricultural University, Urumqi, China
SAC genes have been identified to play a variety of biological functions and responses to various stresses. Previously, SAC genes have been recognized in animals and Arabidopsis. For the very first time, we identified 157 SAC genes in eight cotton species including three diploids and five tetraploids with 23 SAC members in G. hirsutum. Evolutionary analysis classified all cotton SAC gene family members into five distinct groups. Cotton SAC genes showed conserved sequence logos and WGD or segmental duplication. Multiple synteny and collinearity analyses revealed gene family expansion and purifying selection pressure during evolution. G. hirsutum SAC genes showed uneven chromosomal distribution, multiple exons/introns, conserved protein motifs, and various growth and stress-related cis-elements. Expression pattern analysis revealed three GhSAC genes (GhSAC3, GhSAC14, and GhSAC20) preferentially expressed in flower, five genes (GhSAC1, GhSAC6, GhSAC9, GhSAC13, and GhSAC18) preferentially expressed in ovule and one gene (GhSAC5) preferentially expressed in fiber. Similarly, abiotic stress treatment verified that GhSAC5 was downregulated under all stresses, GhSAC6 and GhSAC9 were upregulated under NaCl treatment, and GhSAC9 and GhSAC18 were upregulated under PEG and heat treatment respectively. Overall, this study identified key genes related to flower, ovule, and fiber development and important genetic material for breeding cotton under abiotic stress conditions.
Introduction
Phospholipids group that is different from other phospholipids based on the existence of a phosphate group of phosphatidylinositol (PI) are known as Phosphoinositides. Phosphoinositides exist in seven forms and are important for the release of intracellular calcium and the activation of protein kinase C (Toker, 1998). In animals and yeast (Saccharomyces cerevisiae), phosphoinositides play a key role in biological mechanisms including maintenance of vacuole morphology, actin cytoskeleton organization, vesicle trafficking, regulation of lipid storage, and proteins activation (Takenawa and Itoh, 2001). SAC proteins have been categorized into two groups based on protein sequences except for SAC domains (Hughes et al., 2000). In one group SAC domain is found in N-terminal and is linked to type II 5-phosphatase located in C-terminal. The SAC domain of the second group is associated with the C-terminal with unknown domains. In the second group, protein’s C-terminal regions are different in length from amino acid sequences. SAC domains have seven conserved motifs and about a length of 400 amino acids. SAC domains are important for phosphatase activities (Guo et al., 1999; Hughes et al., 2000).
In plants, SAC genes have been identified to play a variety of functions including pollen tube growth, vesicle trafficking, osmotic regulation, and responses to hormonal treatments and various stresses (Zhong and Ye, 2003; Wang et al., 2017; Xiong et al., 2019). Genome analysis indicated a large number of SAC genes in Arabidopsis. However, limited studies investigated the functions of SAC genes in plants. In Arabidopsis, FRA7 encodes a SAC protein and plays important functions as cell morphogenesis was altered in the fra7 mutant (Erdman et al., 1998). Further, defective cell morphogenesis and cell wall biosynthesis was observed by truncated SAC1 in Arabidopsis (Zhong et al., 2005). Arabidopsis SAC2, SAC3, SAC4, and SAC5 have recognized tonoplast-associated enzymes and play functions in vacuolar morphology. SAC6 showed high expression in flowers with induced expression by salinity stress (Zhong and Ye, 2003), and SAC7 showed involvement in root hair growth in Arabidopsis (Thole et al., 2008).
Cotton is a chief fiber crop and a model to study polyploidy, species evolution, cellulose biosynthesis as well as cell wall development (Senchina et al., 2003). Fiber development is an intricate process that entails several plant hormones such as auxin, gibberellins (GAs), ethylene, and brassinosteroids (Seagull and Giavalis, 2004; Samuel Yang et al., 2006; Ali et al., 2021; Wu et al., 2021a). The genus Gossypium contains about 45 diploid species and seven tetraploid cotton species (Li et al., 2019; Yang et al., 2020). All diploid and tetraploid Gossypium species constitute a single monophyletic group originating from a common ancestor around 5–10 million years ago (mya). Among seven allopolyploid cotton species, including G. hirsutum (AD1), G. barbadense (AD2), G. tomentosum (AD3), G. mustelinum (AD4), G. darwinii (AD5), G. ekmanianum (AD6), and G stephensii (AD7), G. mustelinum may serve as the basal clade, with AD1 and G. tomentosum forming the second clade, whereas AD2 and G. darwinii form the third clade (Huang et al., 2021). Hybridization among A genome having similar genomic characteristics of G. herbaceum (A1) or G. arboreum (A2) and a D genome having similar genomic characteristics of G. raimondii (D5) with subsequent polyploidization gave rise to seven tetraploid cotton species including G. hirsutum and G. barbadense around 1-2 mya (Wendel and Cronn, 2003; Malik et al., 2018). With the improvements in sequencing and assembly of cotton genomes (Paterson et al., 2012; Du et al., 2018) it is possible to perform a complete study of cotton gene families. Functions of many SAC genes have been identified in Arabidopsis but there is no previously published study of SAC genes in cotton.
We comprehensively identified and characterized the SAC gene family members in three diploid species (G. herbaceum, G. arboreum, and G. raimondii), and five tetraploid species (G. hirsutum, G. darwinii, G. tomentosum, G. barbadense, and G. mustelinum) of cotton. The evolutionary relationship among cotton SAC genes was determined by phylogenetic analysis, gene structure, conserved motifs, and sequence logos analysis. Next, multiple synteny analysis and collinearity analysis with nonsynonymous (Ka) and synonymous (Ks) substitution ratios (Ka/Ks ratios) were estimated. Moreover, functions of GhSAC genes were observed by promoter cis-elements analysis, tissue specific expression patterns analysis, and the expression of GhSAC genes after abiotic stress treatments.
Materials and methods
Identification of cotton SAC genes
The gene sequences, protein, cDNA and gene annotation, and genome files (gff) of G. herbaceum (WHU, version 1.0), G. arboreum (ICR, version 1.0), G. raimondii (JGI, version 1.0), G. hirsutum (ICR, ZM24 version 1.0), G. barbadense (HAU, version 1.0), G. tomentosum (HGS, version 1.0), G. mustelinum (HGS, version 1.0) G. darwinii (HGS, version 1.0) were obtained from the CottonFGD database (Zhu et al., 2017). The identified SAC protein sequences in Arabidopsis (Zhong and Ye, 2003) were used to find the SAC genes in observed cotton species by Local BLASTP search. The identified SAC genes were also confirmed by HMM (hidden Markov model) profile obtained from the Pfam (PF02383) database (Finn et al., 2016), PROSITE (PS50275), and Interproscan 63.0 (IPR002013) (http://www.ebi.ac.uk/InterProScan/) (Jones et al., 2014). Identified SAC domains containing proteins were further confirmed by NCBI Batch-CD search (https://www.ncbi.nlm.nih.gov/Structure/bwrpsb/bwrpsb.cgi). We also compared the results of GhSAC genes identified from G. hirsutum (ICR, ZM24 version 1.0) with HAU, JGI, NAU, and ICR (TM-1 version 1.0) and found no difference.
Phylogenetic and sequence logos analysis of SAC genes
For the phylogenetic analysis, amino acid sequences from G. arboreum, G. hirsutum, G. herbaceum, G. raimondii, G. darwinii, G. barbadense, G. mustelinum, and G. tomentosum were aligned by Clustal (Larkin et al., 2007). MEGA 7.0 with ML (Maximum likelihood) method and 1000 bootstrap value was used to generate a tree. For sequence logos analysis, we aligned the SAC protein sequence of G. arboreum, G. hirsutum, G. herbaceum, G. raimondii, G. darwinii, G. barbadense, G. mustelinum, and G. tomentosum by Clustal X 2.0 (Thompson et al., 1997). Sequence logos were constructed using the online tool WEBLOG (Crooks et al., 2004).
Gene structure, motif distribution, and promoter cis-elements analysis
For gene structure analysis, ClustalW was used to align GhSAC protein sequences, and MEGA 7.0 (Kumar et al., 2016) was used to construct an NJ tree. The exon/intron pattern was predicted by GSDS 2.0 (Hu et al., 2010). Protein motif distribution patterns were determined by using the MEME program (http://meme-suite.org/tools/meme) (Bailey et al., 2006) as stated before (Qanmber et al., 2018). Next, for the analysis of cis-elements, 2000 bp promoter sequences of GhSAC were obtained from CottonFGD (Zhu et al., 2017). The GhSAC promoter cis-elements were predicted using the PlantCARE (Plant Cis-Acting Regulatory Element) database (Lescot et al., 2002).
Chromosomal location, gene duplication, and multiple synteny analysis
To investigate the chromosomal location of GhSACs, gff-files of cotton genome annotation data were extracted from the CottonGen database (ftp://ftp.bioinfo.wsu.edu/species/Gossypium_hirsutum/NAU-NBI_G) and genes were mapped by MapInspect software (Jia et al., 2018) on their chromosomes. For gene duplication analysis CIRCOS (Krzywinski et al., 2009) and figure was made by TBtools (Chen et al., 2020). Next, we used PAL2NAL (Suyama et al., 2006) and PAML package (Yang, 2007) to calculate Ka/Ks values.
RNA extraction and qRT-PCR analysis
Leaf samples of various tissues were collected and RNAprep Pure Plant Kit (TianGen, Beijing, China) was used to obtain RNA. RNA was converted into cDNA by EasyScript Allin- First-strand cDNA synthesis SuperMix for RTqPCR kit (TransGen, Beijing, China) and used as a template for qRT-PCR. TransStart Top Green qPCR SuperMix (TransGen, Beijing, China) was used to perform qRT-qPCR in LightCycler 480 (Roche, Basel, Switzerland). Each experiment was conducted in three biological replicates and GhHis3 (AF024716) was used for the normalization of gene expression. Primers used in this study are given in Supplementary Table S1 and qRT-PCR analysis was performed by the 2 −ΔCT method (Livak and Schmittgen, 2001).
Results
Genomic identification of SAC genes
In this study we identified 157 SAC genes in eight Gossypium species including 10 genes in G. herbaceum, 11 genes in G. arboreum, 12 genes in G. raimondii, 23 genes in G. hirsutum, 26 genes in G. mustelinum, 25 genes in G. barbadense, 25 genes in G. tomentosum and 25 genes each in G. darwinii (Supplementary Table S2). Interestingly, D genome cotton G. raimondii contains more numbers of genes than A genome cotton G. arboreum or G. herbaceum. Similarly, tetraploid cotton species (G. barbadense, G. hirsutum, G. mustelinum, G. tomentosum, and G. darwinii) contained almost double the numbers of SAC genes than diploid cotton species (G. arboreum, G. herbaceum, and G. raimondii). Among tetraploid species, G. hirsutum showed fewer numbers of SAC genes, however, indicated the effects of hybridization and polyploidization in allotetraploid cotton species. All identified SAC gene family members were renamed according to the position on their corresponding chromosomes (Supplementary Table S2).
Next, we predicted the basic features of SAC genes in observed Gossypium species and presented them in Table 1. Results indicated that 10 SAC genes of G. herbaceum showed protein length ranges from 558-1631 amino acids (aa) with a mean length of 861.1aa, a median length of 831.5aa and a total length of 8611aa, and 0.0506% occupied a position in the genome. G. arboreum SAC genes showed protein length ranges from 597-1631aa with a mean length of 843.18aa, a median length of 828aa and total length of 9275aa, and 0.0575% occupied position in the genome. G. raimondii SAC genes showed protein length ranges from 188-1631aa with a mean length of 851.83aa, median length of 828.5aa, a total length of 10222aa, and 0.0681% occupied position in the genome. Allotetraploid cotton G. hirsutum SAC genes showed protein length ranges from 565-1631aa with a mean length of 868.17aa, the median length of 828aa, and total length of 19968aa and 0.0673% occupied a position in the genome.
Phylogenetic analysis and sequence logos analysis of SAC gene family
To explore the evolutionary relationship of cotton SAC genes, all protein sequences were subjected to MEGA 7.0 software and a phylogenetic tree was constructed. To indicate the SAC genes from G. arboreum, G. herbaceum, G. raimondii, G. hirsutum, G. barbadense, G. tomentosum, G. mustelinum, and G. darwinii, the prefixes Ga, Ghe, Gr, Gh, Gb, Gt, Gm and Gd were used, respectively. The phylogenetic tree classified cotton SAC genes into five distinct groups SAC a-d (Figure 1). SAC-d and SAC-c were the largest groups containing 44 members each while SAC-e was the smallest with 12 members. SAC-b was the second largest group with 36 members. The phylogenetic tree displayed that most homologous SAC genes between diploids and tetraploids were closely clustered in the same group, indicating the expansion and evolutionary relationship of the SAC gene family. The phylogenetic tree indicated that groups SAC-a, SAC-b, SAC-c, and SAC-d contain SAC genes from eight observed cotton species while SAC-e lacks G. raimondii genes.
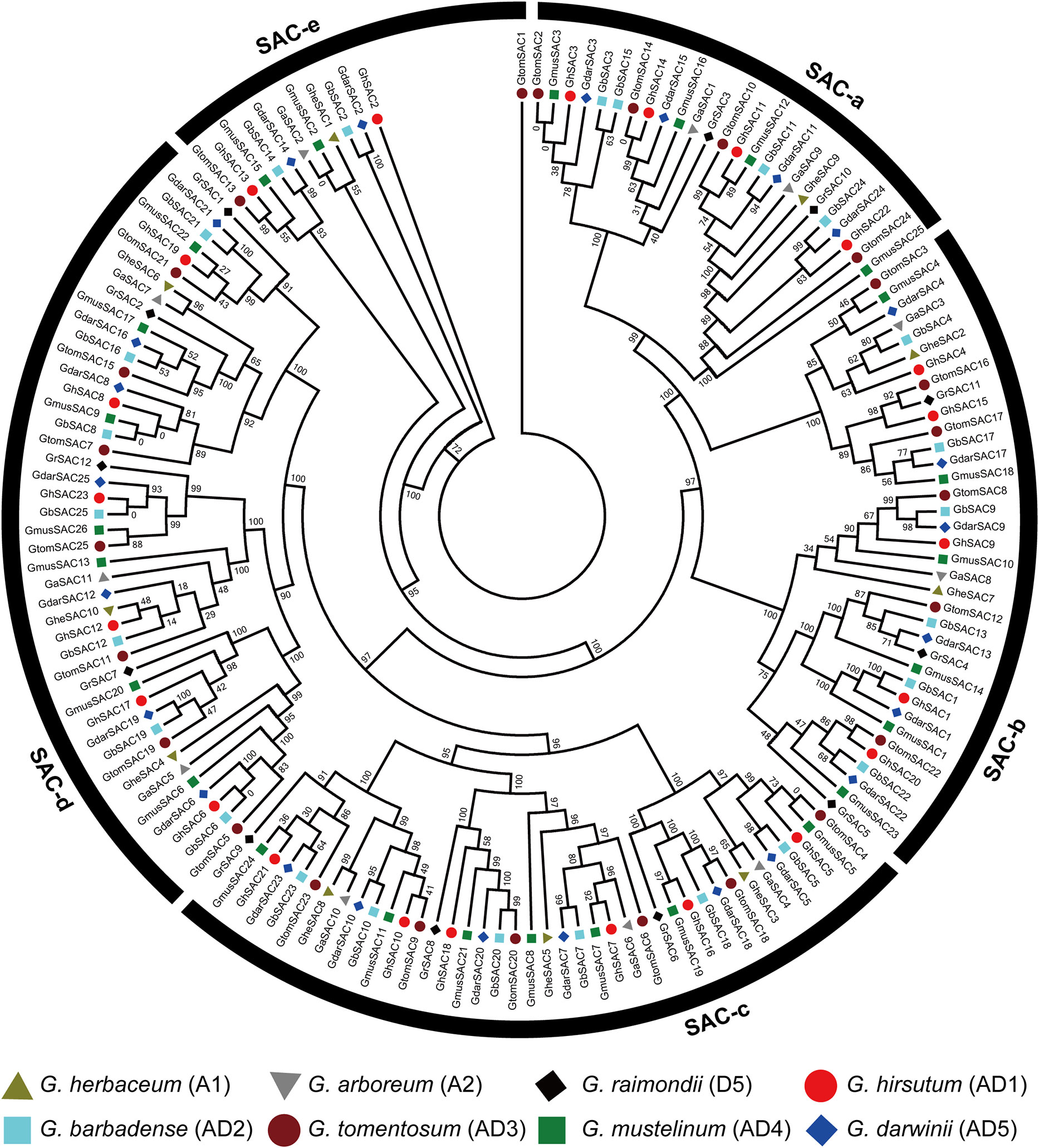
Figure 1 Phylogenetic analysis of cotton SAC genes. Phylogenetic tree among 157 SAC genes from three diploids (G. herbaceum, G. arboreum, and G. raimondii) and five tetraploids (G. hirsutum, G. barbadense, G. tomentosum, G. mustelinum, and G. darwinii) cotton species. The prefixes Ghe. Ga, Gr, Gh, Gb, Gt, Gm, and Gd represents G. herbaceum, G. arboreum, G. raimondii, G. hirsutum, G. barbadense, G. tomentosum, G. mustelinum and G. darwinii respectively.
Further, the evolutionary pattern of SAC genes was observed in eight observed cotton species. Multiple sequence alignment of G. arboreum, G. hirsutum, G. herbaceum, G. raimondii, G. darwinii, G. barbadense, G. mustelinum, and G. tomentosum SAC genes was performed in MEGA 7.0 software and sequence logos were constructed (Supplementary Figure S1). Sequence logos of conserved amino acid residues among all the observed species were highly conserved (Supplementary Figure S1A–H). Sequence logos of conserved amino acid residues provide a better explanation of sequence identity.
Gene duplication, multiple synteny, and collinearity analysis of SAC genes
To study the evolution and effects of hybridization and polyploidization, we identified the types of duplication of SAC genes in observed cotton species. Results identified that G. arboreum, G. hirsutum, G. herbaceum, G. raimondii, G. darwinii, G. barbadense, G. mustelinum, and G. tomentosum SAC genes showed WGD (whole genome duplication) or segmental duplication. However, one SAC gene from G. raimondii, two SAC genes from G. barbadense, two SAC genes from G. tomentosum, one SAC gene from G. mustelinum, and two SAC gene from G. darwinii showed the dispersed type of gene duplication. Additionally, one SAC gene from G. tomentosum and one SAC gene from G. mustelinum showed a singleton type of gene duplication (Supplementary Table S3).
Multiple synteny analysis among G. herbaceum, G. arboreum, G. raimondii, G. hirsutum, G. barbadense, G. tomentosum, G. mustelinum, and G. darwinii SAC genes showed 41 orthologous gene pairs between G. hirsutum and G. arboreum, 43 between G. hirsutum and G. herbaceum, 44 between G. hirsutum, and G. raimondii, 65 between G. hirsutum and G. barbadense, 65 between G. hirsutum and G. darwinii, 64 between G. hirsutum and G. mustelinum, and 66 orthologous gene pairs between G. hirsutum and G. tomentosum (Figure 2; Supplementary Table S4). Further, the nonsynonymous and synonymous substitution ratios (Ka/Ks ratios) were estimated to find the type of selection pressure in these orthologous gene pairs during evolution. All homologous gene pairs between G. hirsutum and G. herbaceum, G. hirsutum and G. arboreum, G. hirsutum and G. darwinii showed Ka/Ks ratios less than 1. While all orthologous gene pairs showed Ka/Ks ratios less than 1 except one gene pair G. hirsutum and G. raimondii, one gene pair G. hirsutum and G. barbadense, one gene pair G. hirsutum and G. mustelinum, and two gene pair G. hirsutum and G. tomentosum (Supplementary Table S4).
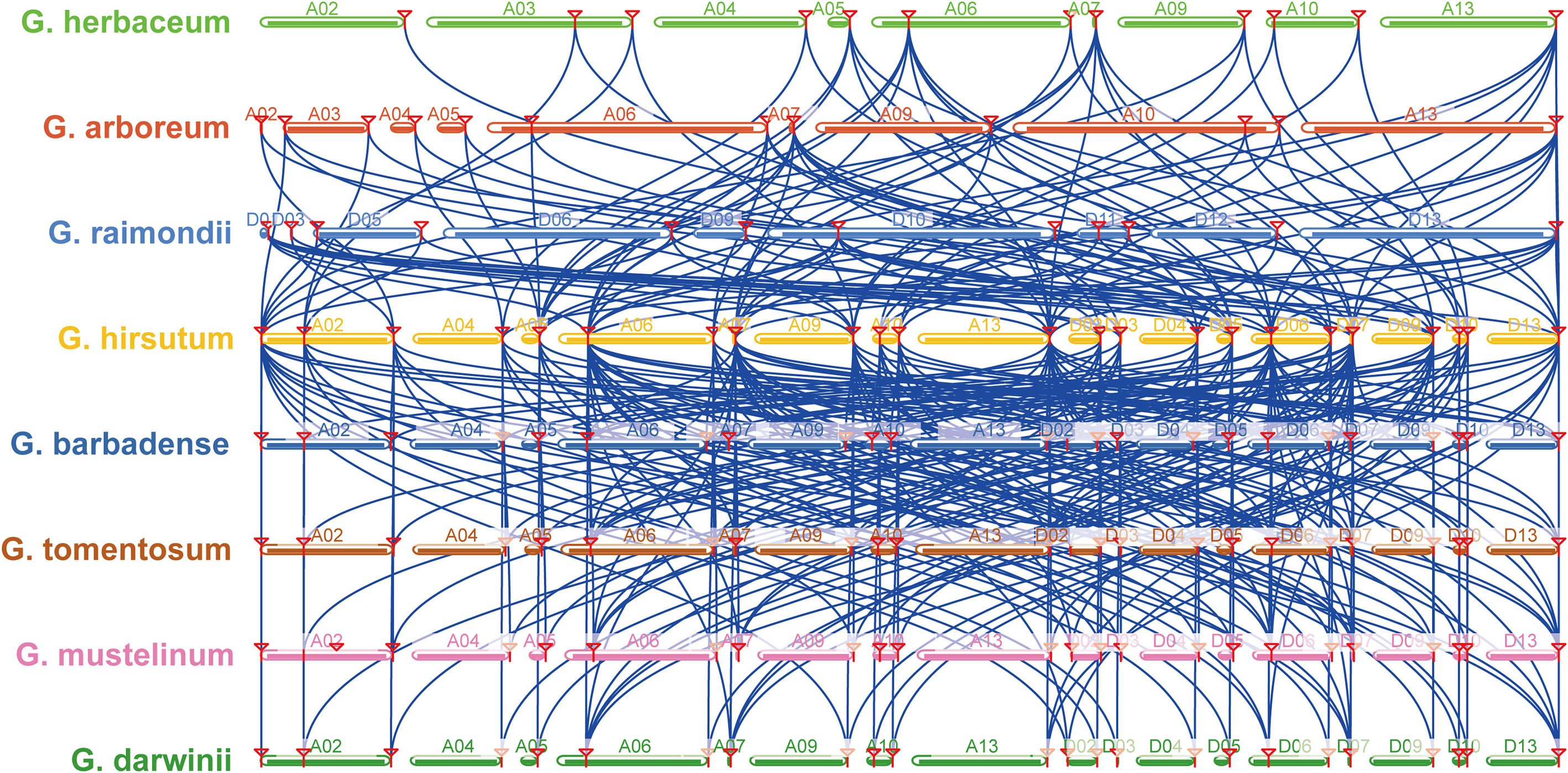
Figure 2 Multiple synteny analysis among cotton SAC genes. Multiple synteny analysis was used to show the orthologous relationship among G. herbaceum, G. arboreum, G. raimondii, G. hirsutum, G. barbadense, G. tomentosum, G. mustelinum, and G. darwinii SAC genes. Chromosomes of different cotton species were represented with different colors.
To explore the locus relationships among the A- and D-subgenomes of G. hirsutum and G. barbadense, we performed a collinearity analysis (Figure 3). A total of 10 orthologous/paralogous pairs were found in G. hirsutum with Ka/Ks < 1 (Figure 3A; Supplementary Table S5). Similarly, a total of 28 orthologous/paralogous gene pairs were found in G. barbadense with Ka/Ks < 1 (Figure 3B; Supplementary Table S5). More precisely, all GhSAC genes showed Ka/Ks values <0.5 while the Ka/Ks values of 22 GbSAC genes were less than 0.5 while five genes showed Ka/Ks values greater than 0.5.
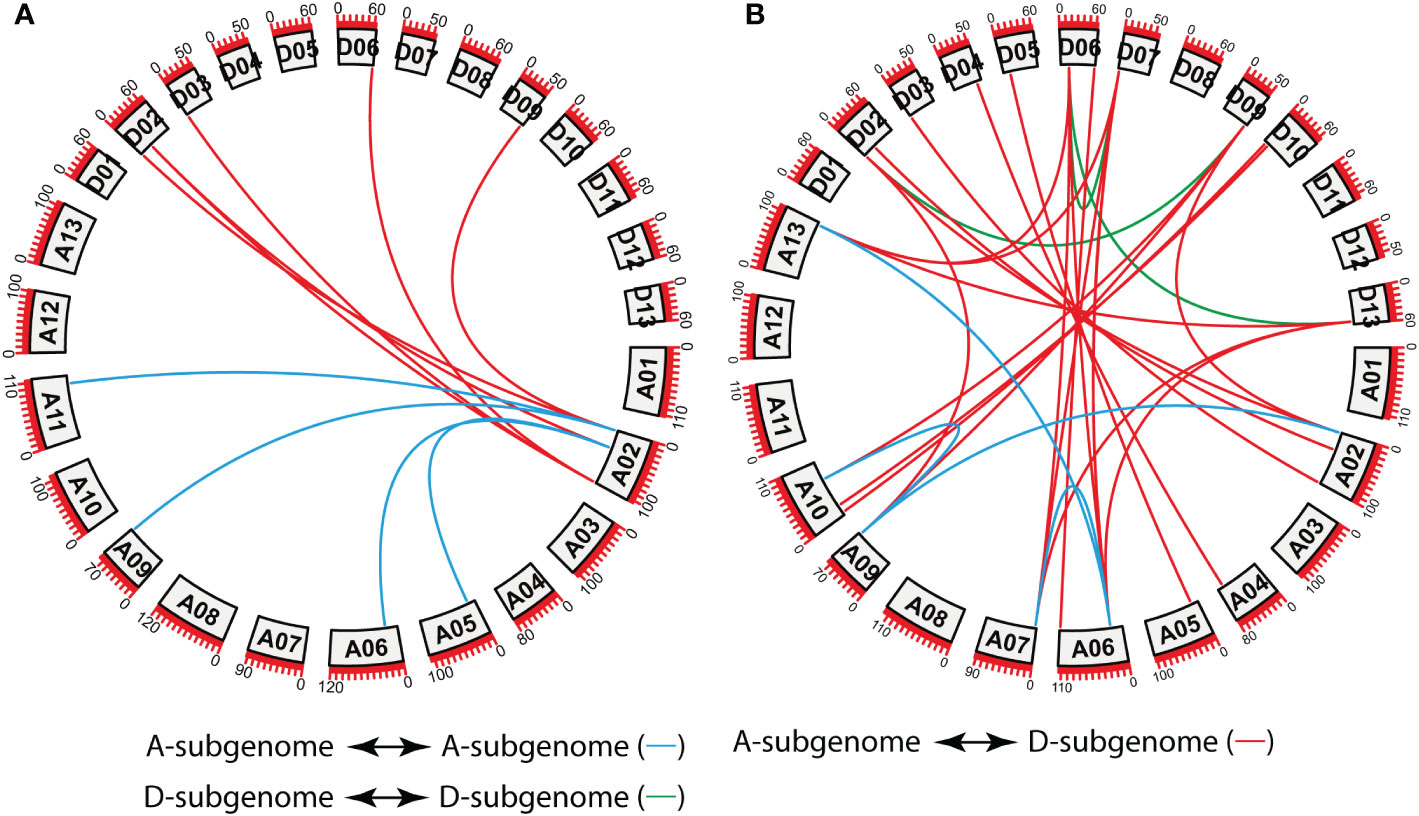
Figure 3 Collinearity analysis of G. hirsutum and G. barbadense SAC genes. (A) Collinearity analysis of G. hirsutum SAC genes. (B) Collinearity analysis of G. barbadense SAC genes. A01 to A13 represents A-subgenome chromosomes while D01 to D13 represents D-subgenome chromosomes. Homologous gene pairs between A- to A-subgenome were represented with blue lines, homologous gene pairs between A- to D-subgenome were represented with red lines, and homologous gene pairs between the D- to D-subgenome were represented with green lines.
Gene structure and protein motif analysis
To study the structural features, exon/intron and the protein motifs of GhSAC family genes were analyzed (Supplementary Figure S2). A NJ phylogenetic tree among GhSAC genes clustered according to the motif distribution pattern and exon-intron structure (Supplementary Figure S2A). The motif distribution pattern indicated the distribution of 10 motifs across the GhSAC proteins. GhSAC proteins with similar motif distribution patterns were closely clustered (Supplementary Figure S2B). Members of the same group have a similar motif distribution pattern, signifying that the motif distribution pattern is highly conserved and they might have identical functions. Next, the gene structure analysis indicated the distribution pattern of CDs, intron, and UTRs. Analysis indicated the presence of multiple introns in all observed GhSAC genes. However, the GhSAC genes with similar CDs, intron, and UTRs structures were found to make a representative clade in the phylogenetic tree (Supplementary Figure S2C).
Chromosomal location and promotor cis-element analysis
Next, we inspected the location of GhSACs on chromosomes (Supplementary Figure S3). Findings showed that 23 GhSAC genes were distributed unevenly on 17 chromosomes. Out of 23 genes, 12 GhSAC genes were placed on the chromosomes of the A-subgenome while 11 GhSAC genes were located on the chromosomes of the D-subgenome. The maximum number of genes (three genes) were allocated on chromosome A02 of the A-subgenome and from D-subgenome the maximum genes were placed on the D10 chromosome (two genes). However, no gene was mapped on chromosome A01, A03, A08, A11 and A12 and D01, D08, D11, and D12 chromosomes.
Furthermore, we used the PlantCARE database to identify the presence of cis-elements controlling the expression of the GhSAC genes. The results revealed that GhSAC gene promoters contain core cis-elements (Figure 4). The GhSAC genes promoter regions shared light-responsive boxes, zein metabolism, circadian control, anaerobic induction, and phytochrome downregulation elements. Further, stress-response elements including low-temperature response elements were present in the GhSAC promotor region. Growth-related elements including meristem expression and endosperm expression, hormone-related elements such as auxin response, salicylic acid response, abscisic acid response, MeJA response, and gibberellin response were found in the GhSAC promotor region.
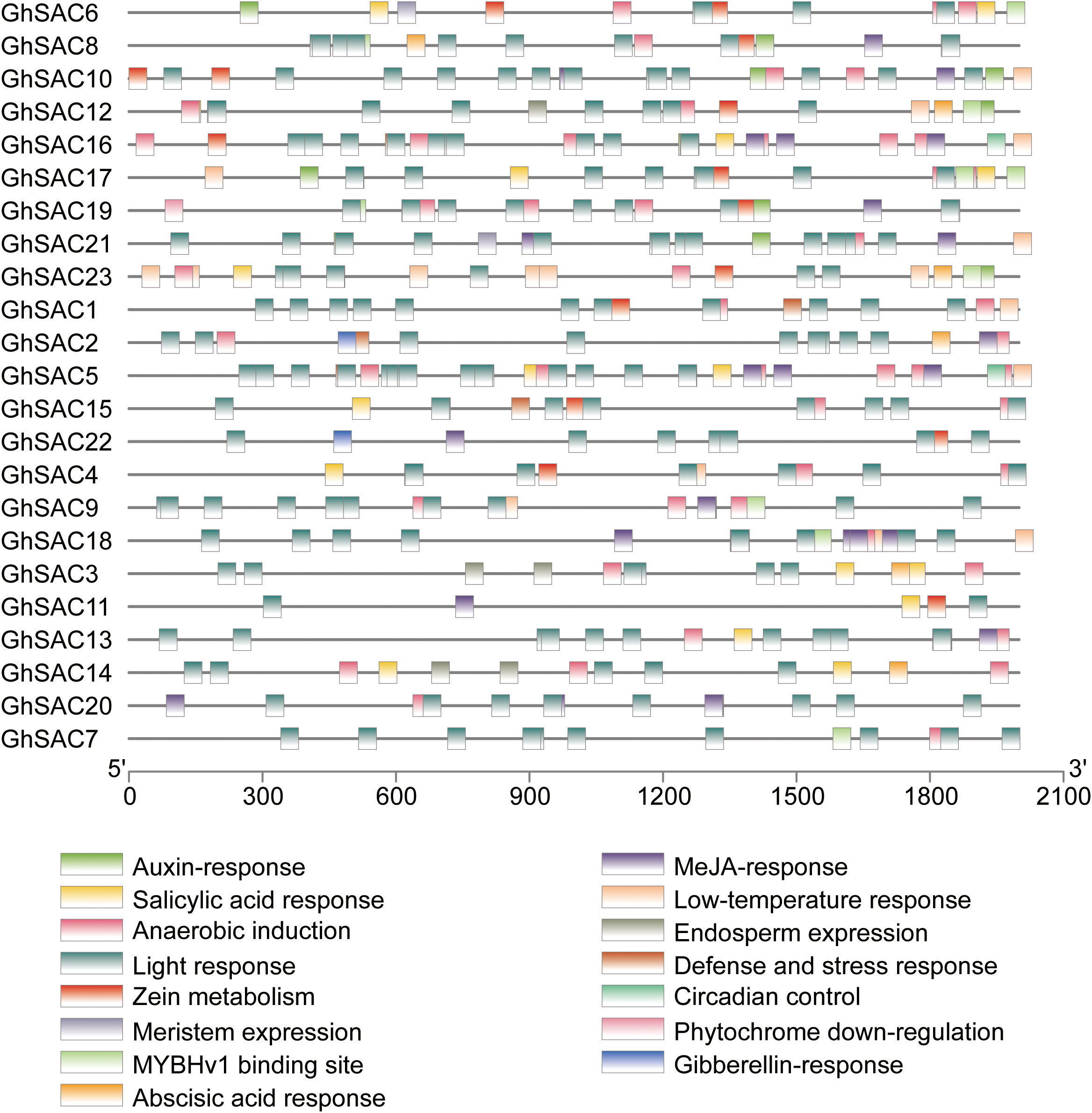
Figure 4 Promoter cis-element analysis of GhSAC genes. G. hirsutum SAC genes promoter region (2kb upstream from start codon) was used to explore cis-elements related to plant growth, abiotic stresses, and phytohormonal responses.
Tissue-specific expression pattern of GhSAC genes
The biological functions of genes are generally correlated with the gene expression pattern. We investigated the transcript level of GhSAC genes in various tissues including root, stem, leaf, flower, -2, 0, 5, 10, 15, 20, and 25 DPA ovule, and 1, 10, 15, 20, and 25 DPA fiber (Figure 5). Results of qRT PCR analysis displayed that nine selected genes showed ubiquitous expression in all observed tissues. More precisely, GhSAC1 showed high enrichment in 15DPA ovule tissues. GhSAC3, GhSAC14, and GhSAC20 were preferentially expressed in flower tissues, and GhSAC5 and GhSAC6 were preferentially expressed in 15DPA fiber and 5DPA ovule. Interestingly, GhSAC9 and GhSAC18 were expressed specifically in the 10DPA ovule. Overall, three GhSAC genes (GhSAC3, GhSAC14, and GhSAC20) showed preferential expression in flower tissues, while five GhSAC genes (GhSAC1, GhSAC6, GhSAC9, GhSAC13, and GhSAC18) showed preferential expression in ovule tissues. However, only one GhSAC gene (GhSAC5) showed preferentially expressed in fiber tissues. From these findings, we may infer that GhSAC genes might play a significant role in flowering and fiber development in cotton.
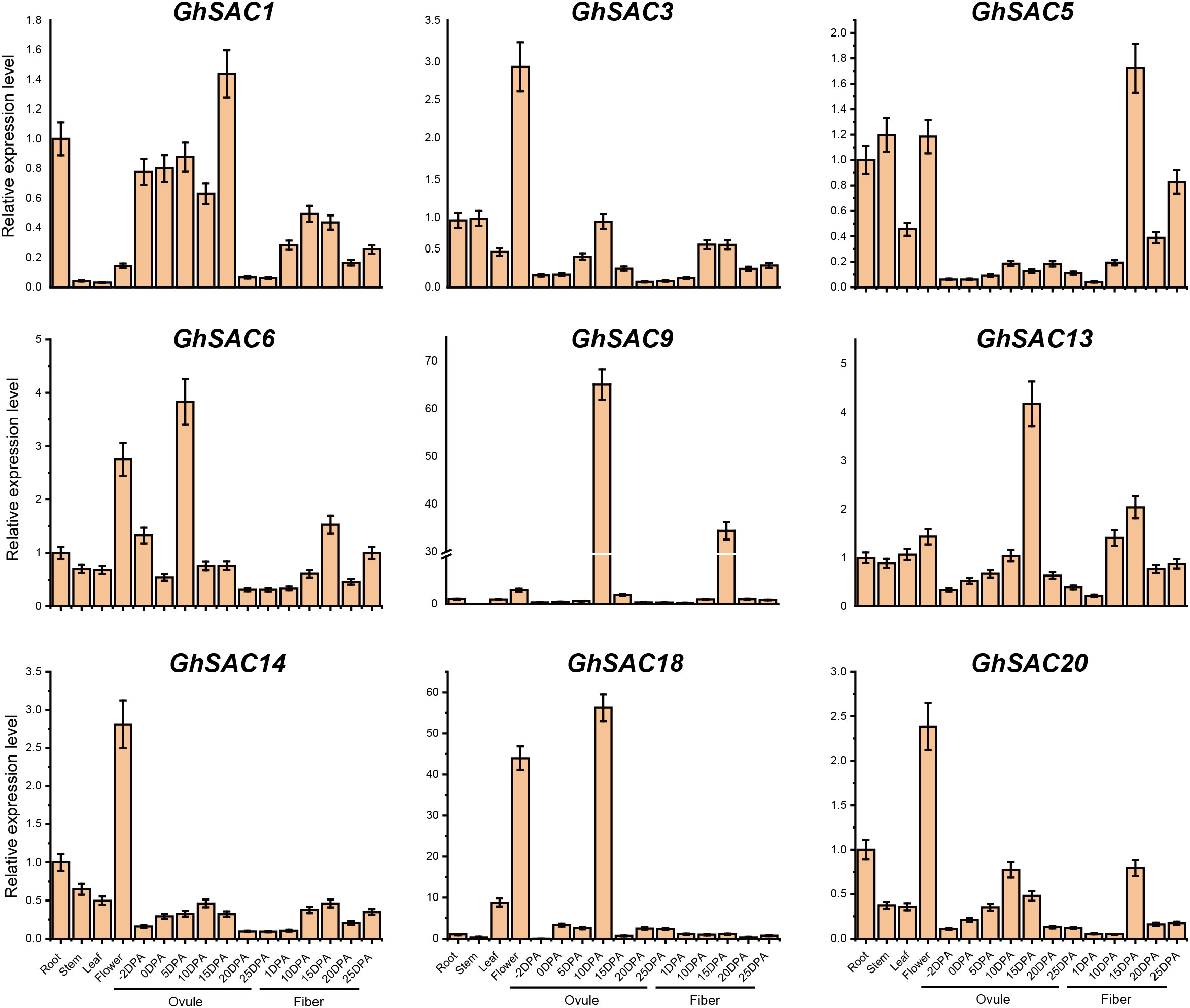
Figure 5 Expression pattern analysis of GhSAC genes. qRT-PCR analysis was performed to observe the relative expression patterns of GhSAC genes in vegetative, ovule, and fiber tissue of the cotton plant. Each experiment was conducted in three biological repeats and the error bar represents the standard deviation among repeats.
Responses of GhSAC genes under abiotic stresses
To check the potential biological and physiological function of GhSAC genes, we performed the tissue specific expression pattern of nine GhSAC genes under various stresses including cold, heat, NaCl, and PEG (Figure 6). Abiotic stresses regulate the expression pattern of various genes and affect plant growth and development. GhSAC genes showed widely variable responses against all stresses. Overall, GhSAC genes showed downregulation under various abiotic stresses except at a few time points for some abiotic stresses. For instance, GhSAC5 was downregulated under all stresses at all time points, while GhSAC1 and GhSAC3 were upregulated only at 1h and 6h after PEG treatment respectively. More precisely, GhSAC6 and GhSAC9 were upregulated under NaCl treatment at all time points, while GhSAC9 and GhSAC18 were upregulated at all time points under PEG and heat stress respectively. However, most of the GhSAC genes did not show any specific pattern of upregulation or downregulation at different time points under any specific abiotic stress treatment. Taken together these findings suggest that the transcript level of GhSAC genes can be regulated by various abiotic stresses illustrating that these might be the possible candidate genes for breeding stress resistance in cotton.
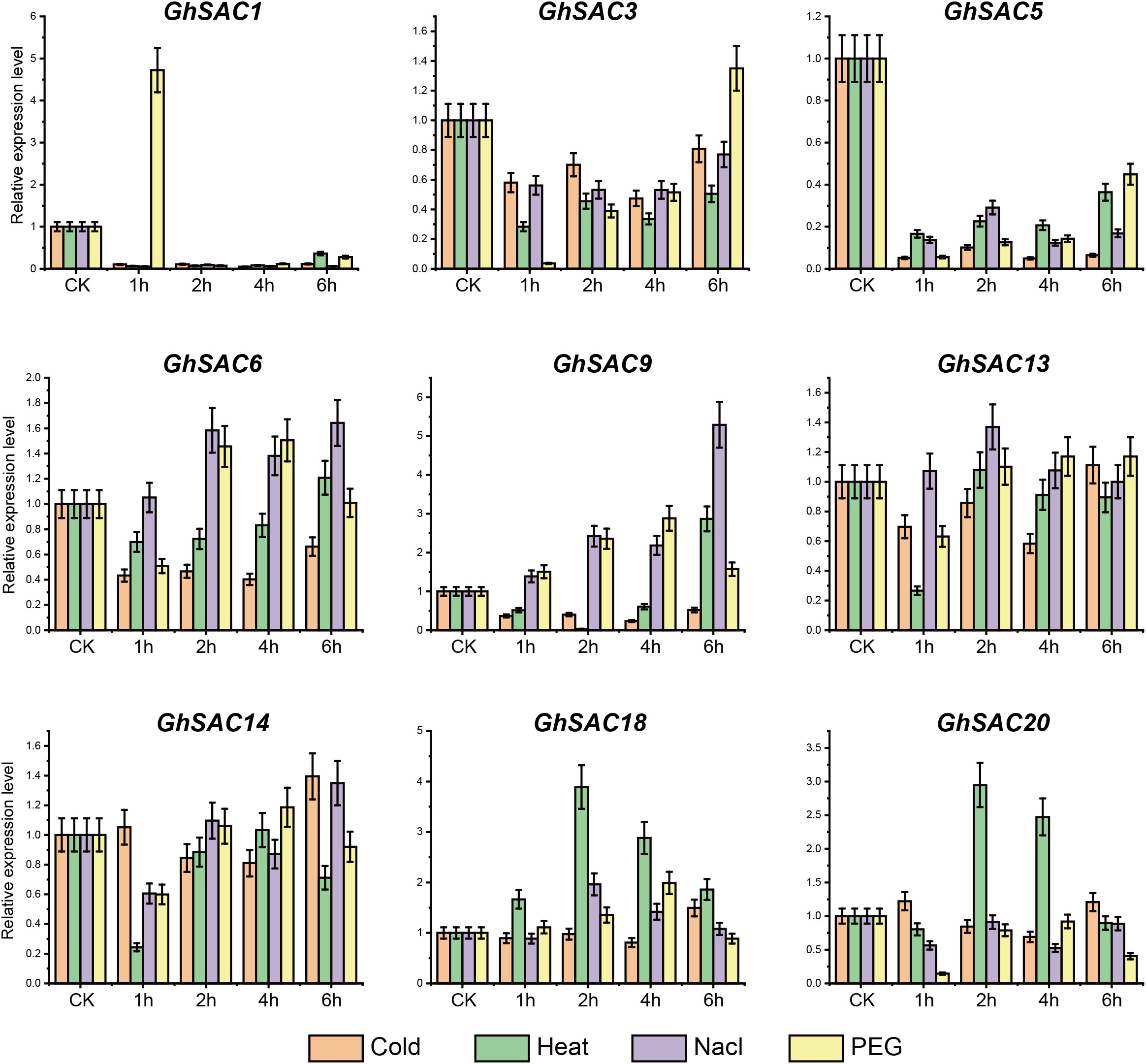
Figure 6 Responses of GhSAC genes under abiotic stresses. qRT-PCR analysis was performed to observe the relative expression patterns of GhSAC genes under cold, heat, NaCl, and PEG treatment. Each experiment was conducted in three biological repeats and the error bar represents the standard deviation among repeats.
Discussion
Allotetraploid cotton including G. hirsutum and G. barbadense are the result of hybridization between A (G. herbaceum or G. arboreum) and D (G. raimondii) genome diploid cotton (Wendel and Cronn, 2003). Availability of cotton genome sequences enabled the researchers to perform the evolutionary and functional analysis of various gene families. Functions of SAC genes have been previously identified in Arabidopsis. SAC genes are essential for the phosphoinositide phosphatase activities in animals and yeast (Zhong and Ye, 2003). But there is no previous study of SAC genes in cotton, especially G. hirsutum. Previously, many gene families including MADS-box (Ren et al., 2017), GhKLCR1 (Li et al., 2019), RH2FE3 (Qanmber et al., 2018), GhGSK (Wang et al., 2018), GhGH3 (Yu et al., 2018), GhBES1 (Liu et al., 2018), GhIDD (Faiza et al., 2019), GhAAI (Qanmber et al., 2019c), GhHH3 (Qanmber et al., 2019a), GhPERK (Qanmber et al., 2019b), GGPPS (Ali et al., 2020), GhGATL (Zheng et al., 2020), GhLOG (Wang et al., 2021), and GhPHD (Wu et al., 2021b) has been studied. In the present study, we conducted a complete investigation of the SAC genes in eight cotton species. Evolutionary relationship through phylogenetic analysis, sequence logos analysis, gene structure, protein motifs, chromosomal localization, gene duplication, multiple synteny, and collinearity analysis was determined. GhSAC gene functions were also observed by cis-element analysis, tissue specific expression pattern analysis, and response of GhSAC genes under abiotic stresses.
Evolution of SAC genes in cotton
SAC genes in eight cotton species including G. arboreum, G. hirsutum, G. herbaceum, G. raimondii, G. darwinii, G. barbadense, G. mustelinum, and G. tomentosum, could be categorized into five groups through phylogenetic analysis. We found that SAC-d was the largest group containing 44 members while SAC–e was the smallest group with 12 members. The phylogenetic tree indicated that all groups namely SAC- a, SAC- b, SAC- c, and SAC- d contained SAC genes from eight observed species while SAC-e lacked the genes from G. raimondii. The presence of SAC genes in each observed species, with the highest number of SACs in G. mustelinum and only 10 SAC genes in G. herbaceum indicates that SAC genes have more expansion in plants. These results were coherent with the sequence logos of G. arboreum, G. hirsutum, G. herbaceum, G. raimondii, G. darwinii, G. barbadense, G. mustelinum, and G. tomentosum that were conserved in all selected Gossypium species, demonstrating that SAC gene family is conserved throughout the evolution. The number of SAC genes in tetraploid species G. hirsutum, G. mustelinum, G. barbadense, G. tomentosum, and G. darwinii was equal to the total of SAC genes in diploid cotton species G. herbaceum, G. arboreum and G. raimondii, which prove that the tetraploid (AD genome) cotton species formed from diploid A- and D-genome ancestors (Wendel, 1989; Wendel and Cronn, 2003).
Structural analysis of GhSAC genes indicated that they have multiple numbers of exons and introns. Structural differences of exon–intron is the result of insertion or deletion and are very important for understanding the evolution of gene families (Lecharny et al., 2003). During evolutions, introns showed weak selection. Loss or gain of introns during eukaryotic diversification was extensive as proved by different genome-wide studies (Rogozin et al., 2003; Roy and Penny, 2007). During the evolution of plant species, introns played a significant role (Roy and Gilbert, 2006). Different length of introns among genes demonstrated their major roles in the functional divergence of GhSAC genes. Further, 10 conserved protein motifs were found in GhSACs with slight protein motif differences that might be related to plant growth and abiotic stress tolerance. Results of protein motif analysis showed the specificity of some motifs to a particular group, signifying the characteristic functions of that group.
Chromosomal location showed that GhSAC genes were distributed unevenly on different chromosomes. Uneven allocation of GhSAC genes on the A and D subgenome chromosomes indicated gene addition or deletion as a result of WGD or segmental duplication events as well as due to incomplete genome sequencing. Most of chromosomes such as A04, A05, A07, A09, A13, D02, D03, D04, D05, D07, D09 and D13 have only one gene. A maximum number of genes (three GhSAC genes) were found on A02 and two GhSAC genes on D06 and D10 chromosomes. Furthermore, GhSAC genes contained various cis-elements in their promotor region related to light responsive, zein metabolism, circadian control, phytochrome regulation elements, anaerobic induction, low temperature, meristem expression, and endosperm expression, auxin response, salicylic acid response, abscisic acid response, MeJA response, and gibberellin response elements. Previous studies found light-induced cis-elements G-box, GT1-motif, I-box, and AT-rich regions (Lam and Chua, 1989; Gilmartin et al., 1992; Foster et al., 1994), auxin-induced cis-elements AuxRE, DR5 (Ulmasov et al., 1997), drought-induced cis-elements CATGTG and CACG (Tran et al., 2004). The existence of different elements in the promotor region of GhSAC genes predicted the functional diversity of GhSAC genes in cotton.
Duplication and expansion of SAC genes
G. hirsutum is used to study polyploidy in plants. Previous studies proved that G. hirsutum was formed by the hybridization of G. arboreum and G. raimondii (Wendel, 1989). During the process of evolution, segmental duplication and translocation are known as chromosome mutation help plants to adapt to environmental stresses (Fraser et al., 2005). In our study, the evolutionary mechanism of SAC genes was not fully revealed by phylogenetic analysis, therefore we explored genomic distribution and duplication. We observed that the total number of GhSAC genes was equal to the total of SAC genes in G. arboreum and G. raimondii. Here, segmental or WGD was the key to SAC gene family extension during evolution. Some previous studies also demonstrated gene family expansion through segmental duplication (Qanmber et al., 2018; Wu et al., 2019; Zhao et al., 2020; Wu et al., 2021b).
In this study, G. arboreum, G. herbaceum, G. raimondii, G. hirsutum, G. barbadense, G. tomentosum, G. mustelinum and G. darwinii SAC genes showed WGD or segmental duplication, however, eight SAC genes with the dispersed type of gene duplication and two SAC genes with singleton type of gene duplication were also observed. Interestingly, GhSAC genes showed only WGD or segmental duplication. Multiple synteny analysis showed almost similar orthologous genes between tetraploid G. hirsutum and diploid G. arboreum, G. herbaceum, and G. raimondii (41, 43 and 44 gene pairs respectively) and between G. hirsutum and G. barbadense, G. tomentosum, G. mustelinum and G. darwinii (65, 65, 64 and 66 gene pairs respectively). Ka/Ks ratios among all orthologous gene pairs were less than one indicating the purifying selection of duplicated genes. Next, the locus relationship among A- subgenomes and D-subgenomes chromosomes of G. hirsutum and G. barbadense showed 10 orthologous/paralogous GhSAC gene pairs in G. hirsutum with Ka/Ks < 1, and 28 orthologous/paralogous genes in G. barbadense with Ka/Ks < 1. The Ka/Ks ratio provides insights into the pressure of selection experienced by duplicated genes during the course of evolution. Ka/Ks = 1.0 represents a neutral selection of duplicated pairs of genes, Ka/Ks < 1.0 exhibits purifying selection, and Ka/Ks > 1.0 shows positive selection during accelerated evolution. Coupled with these findings we summarized that cotton SAC genes experienced WGD or segmental duplication with purifying selection pressure during evolution.
Expression profile analysis of GhSAC genes
Several studies demonstrated that SAC proteins have conserved amino acid motifs essential for the phosphoinositide phosphatase activities in animals and yeast. Gene expression analysis of Arabidopsis SAC proteins verified the differential expression of AtSAC genes in various organs. More specifically, the AtSAC6 gene was primarily expressed in flowers and was highly induced by salinity stress (Zhong and Ye, 2003). Here, the expression level of GhSAC genes was examined in different vegetative and reproductive organs. Expression profile analysis of GhSAC genes displayed that they play important functions in plants. Likewise, Arabidopsis SAC genes, and G. hirsutum SAC genes exhibited differential expression in various organs and tissues. Three GhSAC genes (GhSAC3, GhSAC14, and GhSAC20) showed preferential expression in flower tissues, five GhSAC genes (GhSAC1, GhSAC6, GhSAC9, GhSAC13, and GhSAC18) showed preferential expression in ovule tissues and only one GhSAC gene (GhSAC5) showed preferential expression in fiber tissues. Here, GhSAC6 showed increased transcript levels in all observed tissues and organs specifically high expression in flower and 5DPA ovule. These findings are similar to the previous study as the AtSAC6 gene in Arabidopsis was preferentially expressed in flowers and other organs (Zhong and Ye, 2003). Previous studies of SAC genes in Arabidopsis indicated that AtSAC genes showed lower expression in leaves and roots (Zhong and Ye, 2003), but here we observed that all GhSAC genes showed moderate to low expression levels in leaves and roots. From these findings, we may infer that GhSAC genes might play a vital role in flowering and ovule development in cotton plants.
The cis-elements analysis showed that GhSAC genes can be regulated by abiotic stress and participate in hormone signal transduction, so we validated these results with the help of expression pattern analysis of GhSAC genes in response to different stress stimuli. SAC genes have a key role in the phosphatase activities of animals and yeast (Zhong and Ye, 2003). Phosphoinositides metabolism of plants is mainly regulated by different stress treatments and hormones (Mikami et al., 1998; Meijer et al., 1999; Pical et al., 1999; Meijer et al., 2001). So, we explored the expression patterns of GhSAC genes under abiotic stress treatments. The transcript level of the AtSAC6 was induced by salt stress treatment (Zhong and Ye, 2003) demonstrating that AtSAC6 can be regulated by salt stress. Consistent with the previous studies GhSAC6 showed high expression under salt treatment. Further, hyperosmotic or salt treatment changes the phosphoinositide level in plants (Meijer et al., 1999; Pical et al., 1999; Dewald et al., 2001). Overall, all GhSAC genes showed response under observed abiotic stresses for various time points. For instance, GhSAC5 showed downregulated response under all stresses, while GhSAC1 and GhSAC3 showed upregulated response only at 1h and 6h after PEG treatment respectively. Moreover, GhSAC6 and GhSAC9 showed upregulated response under NaCl treatment, while GhSAC9 and GhSAC18 showed upregulated response under PEG and heat stress treatment respectively. These findings illustrated that the transcript level of GhSACs can be regulated by different abiotic stresses indicating that GhSAC genes can be the possible candidate genes for breeding abiotic stress resistance in cotton.
Conclusion
Here, a total of 157 SAC genes were found in eight species of cotton including 23 genes in G. hirsutum. Based on the phylogenetic tree SAC genes were classified into five distinct groups. WGD or segmental duplication was an important source for the enlargement of the SAC gene family in cotton. Cotton SAC duplicated genes experienced purifying selection pressure and showed conserved amino acid sequence logos during evolution. GhSAC genes showed conserved gene structure with multiple exons/introns and protein motifs. GhSAC genes showed uneven chromosomal distribution patterns on different chromosomes of A- and D-subgenomes. GhSAC genes play essential regulatory roles in the growth of the cotton plant and can be regulated under abiotic stresses. Based on expression patterns, GhSAC genes were associated with flower, ovule, and cotton fiber development. Further, GhSAC genes were regulated by abiotic stresses. For instance, three GhSAC genes showed enrichment in flower tissues, five GhSAC genes were highly expressed in ovule tissues and one GhSAC gene was highly expressed in fiber tissues. Similarly, GhSAC5 was downregulated under all abiotic stresses, GhSAC1 and GhSAC3 were upregulated at 1h and 6h after PEG treatment respectively, GhSAC6 and GhSAC9 were upregulated under NaCl treatment, and GhSAC9 and GhSAC18weres upregulated under PEG and heat stress respectively. Our study provides useful information related to the evolution of the cotton SAC gene family, biological functions of GhSAC genes and laid the foundation for further studies of SAC genes in other plant species.
Data availability statement
The datasets presented in this study can be found in online repositories. The names of the repository/repositories and accession number(s) can be found in the article/Supplementary Material.
Author contributions
MS, LL, SH, GY, and LY conducted the experiments. GQ planned the study and conducted the image analysis. All authors contributed to the article and approved the submitted version.
Funding
This study was supported by the National Natural Science Foundation of China (Project 32001597).
Acknowledgments
The authors would like to thank all study participants in the study.
Conflict of interest
The AE MA declared a shared affiliation with the Authors MS, LL, SH at the time of review.
The remaining authors declare that the research was conducted in the absence of any commercial or financial relationships that could be construed as a potential conflict of interest.
Publisher’s note
All claims expressed in this article are solely those of the authors and do not necessarily represent those of their affiliated organizations, or those of the publisher, the editors and the reviewers. Any product that may be evaluated in this article, or claim that may be made by its manufacturer, is not guaranteed or endorsed by the publisher.
Supplementary material
The Supplementary Material for this article can be found online at: https://www.frontiersin.org/articles/10.3389/fpls.2023.1123745/full#supplementary-material
Supplementary Figure 1 | Sequence logos analysis of cotton SAC genes. Amino acid sequence residue analysis was performed among (A) G. herbaceum, (B) G. arboreum, (C) G. raimondii, (D) G. hirsutum, (E) G. barbadense, (F) G. tomentosum, (G) G. mustelinum and (H) G. darwinii SAC genes across N and C terminals.
Supplementary Figure 2 | Gene structure and protein motif analysis of GhSAC genes. (A) Phylogenetic analysis among GhSAC genes. (B) Protein motifs distribution pattern analysis among GhSAC genes. (C) CDs, introns, and UTR structure of GhSAC genes.
Supplementary Figure 3 | Chromosomal localization of GhSAC genes. GhSAC genes were localized on their corresponding chromosomes and the color of chromosomes represents the gene density on that chromosome. A02 to A13 represent the A-subgenome chromosomes of G. hirsutum and D02 to D13 represent D-subgenome chromosomes of G. hirsutum.
References
Ali, F., Qanmber, G., Li, F., Wang, Z. (2021). Updated role of ABA in seed maturation, dormancy, and germination. J. Adv. Res. 35:199–214. doi: 10.1016/j.jare.2021.03.011
Ali, F., Qanmber, G., Wei, Z., Yu, D., Li, Y., Gan, L., et al. (2020). Genome-wide characterization and expression analysis of geranyl geranyl diphosphate synthase genes of cotton (Gossypium spp.) in plant development and abiotic stresses. BMC Genomics 21:1–15. doi: 10.1186/s12864-020-06970-8
Bailey, T. L., Williams, N., Misleh, C., Li, W. W. (2006). MEME: Discovering and analyzing DNA and protein sequence motifs. Nucleic Acids Res. 34, W369–W373. doi: 10.1093/nar/gkl198
Chen, C., Chen, H., Zhang, Y., Thomas, H. R., Frank, M. H., He, Y., et al. (2020). TBtools: An integrative toolkit developed for interactive analyses of big biological data. Mol. Plant 13, 1194–1202. doi: 10.1016/j.molp.2020.06.009
Crooks, G. E., Hon, G., Chandonia, J.-M., Brenner, S. E. (2004). WebLogo: A sequence logo generator. Genome Res. 14, 1188–1190. doi: 10.1101/gr.849004
Dewald, D. B., Torabinejad, J., Jones, C. A., Shope, J. C., Cangelosi, A. R., Thompson, J. E., et al. (2001). Rapid accumulation of phosphatidylinositol 4, 5-bisphosphate and inositol 1, 4, 5-trisphosphate correlates with calcium mobilization in salt-stressed arabidopsis. Plant Physiol. 126, 759–769. doi: 10.1104/pp.126.2.759
Du, X., Huang, G., He, S., Yang, Z., Sun, G., Ma, X., et al. (2018). Resequencing of 243 diploid cotton accessions based on an updated a genome identifies the genetic basis of key agronomic traits. Nat. Genet. 50, 796–802. doi: 10.1038/s41588-018-0116-x
Erdman, S., Lin, L., Malczynski, M., Snyder, M. (1998). Pheromone-regulated genes required for yeast mating differentiation. J. Cell Biol. 140, 461–483. doi: 10.1083/jcb.140.3.461
Faiza, A., Qanmber, G., Yonghui, L., Shuya, M., Lili, L., Zuoren, Y., et al. (2019). Genome-wide identification of gossypium INDETERMINATE DOMAIN genes and their expression profiles in ovule development and abiotic stress responses. J. Cotton Res. 2, 1–16. doi: 10.1186/s42397-019-0021-6
Finn, R. D., Coggill, P., Eberhardt, R. Y., Eddy, S. R., Mistry, J., Mitchell, A. L., et al. (2016). The pfam protein families database: Towards a more sustainable future. Nucleic Acids Res. 44, D279–D285. doi: 10.1093/nar/gkv1344
Foster, R., Izawa, T., Chua, N. H. (1994). Plant bZIP proteins gather at ACGT elements. FASEB J. 8, 192–200. doi: 10.1096/fasebj.8.2.8119490
Fraser, J. A., Huang, J. C., Pukkila-Worley, R., Alspaugh, J. A., Mitchell, T. G., Heitman, J. (2005). Chromosomal translocation and segmental duplication in cryptococcus neoformans. Eukaryotic Cell 4, 401–406. doi: 10.1128/EC.4.2.401-406.2005
Gilmartin, P. M., Memelink, J., Hiratsuka, K., Kay, S. A., Chua, N.-H. (1992). Characterization of a gene encoding a DNA binding protein with specificity for a light-responsive element. Plant Cell 4, 839–849. doi: 10.1105/tpc.4.7.839
Guo, S., Stolz, L. E., Lemrow, S. M., York, J. D. (1999). SAC1-like domains of yeast SAC1, INP52, and INP53 and of human synaptojanin encode polyphosphoinositide phosphatases. J. Biol. Chem. 274, 12990–12995. doi: 10.1074/jbc.274.19.12990
Huang, G., Huang, J.-Q., Chen, X.-Y., Zhu, Y.-X. (2021). Recent advances and future perspectives in cotton research. Annu. Rev. Plant Biol. 72, 437–462. doi: 10.1146/annurev-arplant-080720-113241
Hughes, W. E., Cooke, F. T., Parker, P. J. (2000). Sac phosphatase domain proteins. Biochem. J. 350, 337–352. doi: 10.1042/bj3500337
Hu, S., Liu, D., Wang, C., Chen, Y., Guo. Borgna, A., Yang, Y. (2010). Liquid-phase epoxidation of trans-stilbene and cis-cyclooctene over vanadium-exchanged faujasite zeolite catalysts. Applied Catalysis A: General. 386, 74–82. doi: 10.1016/j.apcata.2010.07.028
Jia, J., Zhao, P., Cheng, L., Yuan, G., Yang, W., Liu, S., et al. (2018). MADS-box family genes in sheepgrass and their involvement in abiotic stress responses. BMC Plant Biol. 18, 1–11. doi: 10.1186/s12870-018-1259-8
Jones, P., Binns, D., Chang, H.-Y., Fraser, M., Li, W., Mcanulla, C., et al. (2014). InterProScan 5: genome-scale protein function classification. Bioinformatics 30, 1236–1240. doi: 10.1093/bioinformatics/btu031
Krzywinski, M., Schein, J., Birol, I., Connors, J., Gascoyne, R., Horsman, D., et al. (2009). Circos: An information aesthetic for comparative genomics. Genome Res. 19, 1639–1645. doi: 10.1101/gr.092759.109
Kumar, S., Stecher, G., Tamura, K. (2016). MEGA7: molecular evolutionary genetics analysis version 7.0 for bigger datasets. Mol. Biol. Evol. 33, 1870–1874. doi: 10.1093/molbev/msw054
Lam, E., Chua, N.-H. (1989). ASF-2: A factor that binds to the cauliflower mosaic virus 35S promoter and a conserved GATA motif in cab promoters. Plant Cell 1, 1147–1156. doi: 10.1105/tpc.1.12.1147
Larkin, M. A., Blackshields, G., Brown, N. P., Chenna, R., Mcgettigan, P. A., Mcwilliam, H., et al. (2007). Clustal W and clustal X version 2.0. Bioinformatics 23, 2947–2948. doi: 10.1093/bioinformatics/btm404
Lecharny, A., Boudet, N., Gy, I., Aubourg, S., Kreis, M. (2003). Introns in, introns out in plant gene families: A genomic approach of the dynamics of gene structure. J. Struct. Funct. Genomics 3, 111–116. doi: 10.1023/A:1022614001371
Lescot, M., Déhais, P., Thijs, G., Marchal, K., Moreau, Y., Van De Peer, Y., et al. (2002). PlantCARE, a database of plant cis-acting regulatory elements and a portal to tools for in silico analysis of promoter sequences. Nucleic Acids Res. 30, 325–327. doi: 10.1093/nar/30.1.325
Liu, Z., Qanmber, G., Lu, L., Qin, W., Liu, J., Li, J., et al. (2018). Genome-wide analysis of BES1 genes in gossypium revealed their evolutionary conserved roles in brassinosteroid signaling. Sci. China Life Sci. 61, 1566–1582. doi: 10.1007/s11427-018-9412-x
Livak, K. J., Schmittgen, T. D. (2001). Analysis of relative gene expression data using real-time quantitative PCR and the 2– ΔΔCT method. methods 25, 402–408. doi: 10.1006/meth.2001.1262
Li, J., Yu, D., Qanmber, G., Lu, L., Wang, L., Zheng, L., et al. (2019). GhKLCR1, a kinesin light chain-related gene, induces drought-stress sensitivity in arabidopsis. Sci. China Life Sci. 62, 63–75. doi: 10.1007/s11427-018-9307-y
Malik, W., Shah, M. S. A., Abid, M. A., Qanmber, G., Noor, E., Qayyum, A., et al. (2018). Genetic basis of variation for fiber quality and quality related biochemical traits in bt and non-bt colored cotton. Intl. J. Agric. Biol. 20, 2117–2124. doi: 10.17957/IJAB/15.0761
Meijer, H. J., Berrie, C. P., Iurisci, C., Divecha, N., Musgrave, A., Munnik, T. (2001). Identification of a new polyphosphoinositide in plants, phosphatidylinositol 5-monophosphate (PtdIns5P), and its accumulation upon osmotic stress. Biochem. J. 360, 491–498. doi: 10.1042/bj3600491
Meijer, H. J., Divecha, N., Van Den Ende, H., Musgrave, A., Munnik, T. (1999). Hyperosmotic stress induces rapid synthesis of phosphatidyl-d-inositol 3, 5-bisphosphate in plant cells. Planta 208, 294–298. doi: 10.1007/s004250050561
Mikami, K., Katagiri, T., Iuchi, S., Yamaguchi-Shinozaki, K., Shinozaki, K. (1998). A gene encoding phosphatidylinositol-4-phosphate 5-kinase is induced by water stress and abscisic acid in arabidopsis thaliana. Plant J. 15, 563–568. doi: 10.1046/j.1365-313X.1998.00227.x
Paterson, A. H., Wendel, J. F., Gundlach, H., Guo, H., Jenkins, J., Jin, D., et al. (2012). Repeated polyploidization of gossypium genomes and the evolution of spinnable cotton fibres. Nature 492, 423–427. doi: 10.1038/nature11798
Pical, C., Westergren, T., Dove, S. K., Larsson, C., Sommarin, M. (1999). Salinity and hyperosmotic stress induce rapid increases in phosphatidylinositol 4, 5-bisphosphate, diacylglycerol pyrophosphate, and phosphatidylcholine in arabidopsis thaliana cells. J. Biol. Chem. 274, 38232–38240. doi: 10.1074/jbc.274.53.38232
Qanmber, G., Ali, F., Lu, L., Mo, H., Ma, S., Wang, Z., et al. (2019a). Identification of histone H3 (HH3) genes in gossypium hirsutum revealed diverse expression during ovule development and stress responses. Genes 10, 355. doi: 10.3390/genes10050355
Qanmber, G., Daoqian, Y., Jie, L., Lingling, W., Shuya, M., Lili, L., et al. (2018). Genome-wide identification and expression analysis of gossypium RING-H2 finger E3 ligase genes revealed their roles in fiber development, and phytohormone and abiotic stress responses. J. Cotton Res. 1, 1–17. doi: 10.1186/s42397-018-0004-z
Qanmber, G., Liu, J., Yu, D., Liu, Z., Lu, L., Mo, H., et al. (2019b). Genome-wide identification and characterization of the PERK gene family in gossypium hirsutum reveals gene duplication and functional divergence. Int. J. Mol. Sci. 20, 1750. doi: 10.3390/ijms20071750
Qanmber, G., Lu, L., Liu, Z., Yu, D., Zhou, K., Huo, P., et al. (2019c). Genome-wide identification of GhAAI genes reveals that GhAAI66 triggers a phase transition to induce early flowering. J. Exp. Bot. 70, 4721–4736. doi: 10.1093/jxb/erz239
Ren, Z., Yu, D., Yang, Z., Li, C., Qanmber, G., Li, Y., et al. (2017). Genome-wide identification of the MIKC-type MADS-box gene family in gossypium hirsutum l. unravels their roles in flowering. Front. Plant Sci. 8, 384. doi: 10.3389/fpls.2017.00384
Rogozin, I. B., Wolf, Y. I., Sorokin, A. V., Mirkin, B. G., Koonin, E. V. (2003). Remarkable interkingdom conservation of intron positions and massive, lineage-specific intron loss and gain in eukaryotic evolution. Curr. Biol. 13, 1512–1517. doi: 10.1016/S0960-9822(03)00558-X
Roy, S. W., Gilbert, W. (2006). The evolution of spliceosomal introns: patterns, puzzles and progress. Nat. Rev. Genet. 7, 211–221. doi: 10.1038/nrg1807
Roy, S. W., Penny, D. (2007). Patterns of intron loss and gain in plants: intron loss–dominated evolution and genome-wide comparison of o. sativa and a. thaliana. Mol. Biol. Evol. 24, 171–181. doi: 10.1093/molbev/msl159
Samuel Yang, S., Cheung, F., Lee, J. J., Ha, M., Wei, N. E., Sze, S. H., et al. (2006). Accumulation of genome-specific transcripts, transcription factors and phytohormonal regulators during early stages of fiber cell development in allotetraploid cotton. Plant J. 47, 761–775. doi: 10.1111/j.1365-313X.2006.02829.x
Seagull, R., Giavalis, S. (2004). Pre-and post-anthesis application of exogenous hormones alters fiber production in gossypium hirsutum l. cultivar maxxa GTO. J. cotton sci 8:105–111.
Senchina, D. S., Alvarez, I., Cronn, R. C., Liu, B., Rong, J., Noyes, R. D., et al. (2003). Rate variation among nuclear genes and the age of polyploidy in gossypium. Mol. Biol. Evol. 20, 633–643. doi: 10.1093/molbev/msg065
Suyama, M., Torrents, D., Bork, P. (2006). PAL2NAL: Robust conversion of protein sequence alignments into the corresponding codon alignments. Nucleic Acids Res. 34, W609–W612. doi: 10.1093/nar/gkl315
Takenawa, T., Itoh, T. (2001). Phosphoinositides, key molecules for regulation of actin cytoskeletal organization and membrane traffic from the plasma membrane. Biochim. Biophys. Acta (BBA)-Molecular Cell Biol. Lipids 1533, 190–206. doi: 10.1016/s1388-1981(01)00165-2
Thole, J. M., Vermeer, J. E., Zhang, Y., Gadella, T. W., Nielsen, E. (2008). Root hair defective4 encodes a phosphatidylinositol-4-phosphate phosphatase required for proper root hair development in arabidopsis thaliana. Plant Cell 20, 381–395. doi: 10.1105/tpc.107.054304
Thompson, J. D., Gibson, T. J., Plewniak, F., Jeanmougin, F., Higgins, D. G. (1997). The CLUSTAL_X windows interface: Flexible strategies for multiple sequence alignment aided by quality analysis tools. Nucleic Acids Res. 25, 4876–4882. doi: 10.1093/nar/25.24.4876
Toker, A. (1998). The synthesis and cellular roles of phosphatidylinositol 4, 5-bisphosphate. Curr. Opin. Cell Biol. 10, 254–261. doi: 10.1016/S0955-0674(98)80148-8
Tran, L.-S. P., Nakashima, K., Sakuma, Y., Simpson, S. D., Fujita, Y., Maruyama, K., et al. (2004). Isolation and functional analysis of arabidopsis stress-inducible NAC transcription factors that bind to a drought-responsive cis-element in the early responsive to dehydration stress 1 promoter. Plant Cell 16, 2481–2498. doi: 10.1105/tpc.104.022699
Ulmasov, T., Murfett, J., Hagen, G., Guilfoyle, T. J. (1997). Aux/IAA proteins repress expression of reporter genes containing natural and highly active synthetic auxin response elements. Plant Cell 9, 1963–1971. doi: 10.1105/tpc.9.11.1963
Wang, L., Hu, Z., Zhu, M., Zhu, Z., Hu, J., Qanmber, G., et al. (2017). The abiotic stress-responsive NAC transcription factor SlNAC11 is involved in drought and salt response in tomato (Solanum lycopersicum l.). Plant Cell Tissue Organ Culture (PCTOC) 129, 161–174. doi: 10.1007/s11240-017-1167-x
Wang, R., Liu, L., Kong, Z., Li, S., Lu, L., Chen, G., et al. (2021). Identification of GhLOG gene family revealed that GhLOG3 is involved in regulating salinity tolerance in cotton (Gossypium hirsutum l.). Plant Physiol. Biochem 166:328–340. doi: 10.1016/j.plaphy.2021.06.011
Wang, L., Yang, Z., Zhang, B., Yu, D., Liu, J., Gong, Q., et al. (2018). Genome-wide characterization and phylogenetic analysis of GSK gene family in three species of cotton: Evidence for a role of some GSKs in fiber development and responses to stress. BMC Plant Biol. 18, 1–21. doi: 10.1186/s12870-018-1526-8
Wendel, J. F. (1989). New world tetraploid cottons contain old world cytoplasm. Proc. Natl. Acad. Sci. 86, 4132–4136. doi: 10.1073/pnas.86.11.4132
Wendel, J. F., Cronn, R. C. (2003). Polyploidy and the evolutionary history of cotton. Adv. Agron. 78, 139. doi: 10.1016/S0065-2113(02)78004-8
Wu, A., Hao, P., Wei, H., Sun, H., Cheng, S., Chen, P., et al. (2019). Genome-wide identification and characterization of glycosyltransferase family 47 in cotton. Front. Genet. 10, 824. doi: 10.3389/fgene.2019.00824
Wu, H., Ren, Z., Zheng, L., Guo, M., Yang, J., Hou, L., et al. (2021a). The bHLH transcription factor GhPAS1 mediates BR signaling to regulate plant development and architecture in cotton. Crop J 9:1049–1059. doi: 10.1016/j.cj.2020.10.014
Wu, H., Zheng, L., Qanmber, G., Guo, M., Wang, Z., Yang, Z. (2021b). Response of phytohormone mediated plant homeodomain (PHD) family to abiotic stress in upland cotton (Gossypium hirsutum spp.). BMC Plant Biol. 21, 1–20. doi: 10.1186/s12870-020-02787-5
Xiong, F., Zhuo, F., Reiter, R. J., Wang, L., Wei, Z., Deng, K., et al. (2019). Hypocotyl elongation inhibition of melatonin is involved in repressing brassinosteroid biosynthesis in arabidopsis. Front. Plant Sci. 10, 1082. doi: 10.3389/fpls.2019.01082
Yang, Z. (2007). PAML 4: Phylogenetic analysis by maximum likelihood. Mol. Biol. Evol. 24, 1586–1591. doi: 10.1093/molbev/msm088
Yang, Z., Qanmber, G., Wang, Z., Yang, Z., Li, F. (2020). Gossypium genomics: Trends, scope, and utilization for cotton improvement. Trends Plant Sci. 25, 488–500. doi: 10.1016/j.tplants.2019.12.011
Yu, D., Qanmber, G., Lu, L., Wang, L., Li, J., Yang, Z., et al. (2018). Genome-wide analysis of cotton GH3 subfamily II reveals functional divergence in fiber development, hormone response and plant architecture. BMC Plant Biol. 18, 1–18. doi: 10.1186/s12870-018-1545-5
Zhao, L., Lü, Y., Chen, W., Yao, J., Li, Y., Li, Q., et al. (2020). Genome-wide identification and analyses of the AHL gene family in cotton (Gossypium). BMC Genomics 21, 69. doi: 10.1186/s12864-019-6406-6
Zheng, L., Wu, H., Qanmber, G., Ali, F., Wang, L., Liu, Z., et al. (2020). Genome-wide study of the GATL gene family in gossypium hirsutum l. reveals that GhGATL genes act on pectin synthesis to regulate plant growth and fiber elongation. Genes 11, 64. doi: 10.3390/genes11010064
Zhong, R., Burk, D. H., Nairn, C. J., Wood-Jones, A., Morrison, W. H., Ye, Z.-H. (2005). Mutation of SAC1, an arabidopsis SAC domain phosphoinositide phosphatase, causes alterations in cell morphogenesis, cell wall synthesis, and actin organization. Plant Cell 17, 1449–1466. doi: 10.1105/tpc.105.031377
Zhong, R., Ye, Z.-H. (2003). The SAC domain-containing protein gene family in arabidopsis. Plant Physiol. 132, 544–555. doi: 10.1104/pp.103.021444
Keywords: cotton, phylogenetic analysis, SAC genes, multiple synteny, flower, ovule, abiotic stresses
Citation: Shuya M, Le L, Huiyun S, Yu G, Yujun L and Qanmber G (2023) Genomic identification of cotton SAC genes branded ovule and stress-related key genes in Gossypium hirsutum. Front. Plant Sci. 14:1123745. doi: 10.3389/fpls.2023.1123745
Received: 14 December 2022; Accepted: 25 January 2023;
Published: 03 February 2023.
Edited by:
Muhammad Ali Abid, Agricultural Genomics Institute at Shenzhen, Chinese Academy of Agricultural Sciences (CAAS), ChinaReviewed by:
Muhammad Azhar Nadeem, Sivas University of Science and Technology, TürkiyeMuhammad Amir Zia, National Institute for Genomics and Advanced Biotechnology (NIGAB), Pakistan
Copyright © 2023 Shuya, Le, Huiyun, Yu, Yujun and Qanmber. This is an open-access article distributed under the terms of the Creative Commons Attribution License (CC BY). The use, distribution or reproduction in other forums is permitted, provided the original author(s) and the copyright owner(s) are credited and that the original publication in this journal is cited, in accordance with accepted academic practice. No use, distribution or reproduction is permitted which does not comply with these terms.
*Correspondence: Ghulam Qanmber, gqkhan12@gmail.com