- 1Department of Genetics and Biotechnology (DGB), University of Trás-os-Montes e Alto Douro (UTAD), Vila Real, Portugal
- 2Centre for the Research and Technology of Agro-Environmental and Biological Sciences (CITAB), University of Trás-os-Montes e Alto Douro, Vila Real, Portugal
- 3Institute for Innovation, Capacity Building and Sustainability of Agri-food Production (Inov4Agro), University of Trás-os-Montes e Alto Douro, Vila Real, Portugal
- 4Instituto de Biotecnología Vegetal, Universidad Politécnica de Cartagena, Cartagena, Spain
- 5Department of Biology and Environment (DeBA), University of Trás-os-Montes e Alto Douro, Vila Real, Portugal
Several fleshy fruits are highly affected by cracking, a severe physiological disorder that compromises their quality and causes high economical losses to the producers. Cracking can occur due to physiological, genetic or environmental factors and may happen during fruit growth, development and ripening. Moreover, in fleshy fruits, exocarp plays an important role, acting as a mechanical protective barrier, defending against biotic or abiotic factors. Thus, when biochemical properties of the cuticle + epidermis + hypodermis are affected, cracks appear in the fruit skin. The identification of genes involved in development such as cell wall modifications, biosynthesis and transport of cuticular waxes, cuticular membrane deposition and associated transcription factors provides new insights to better understand how fruit cracking is affected by genetic factors. Amongst the major environmental stresses causing cracking are excessive water during fruit development, leading to imbalances in cations such as Ca. This review focus on expression of key genes in these pathways, in their influence in affected fruits and the potential for molecular breeding programs, aiming to develop cultivars more resistant to cracking under adverse environmental conditions.
Introduction
Fruit cracking is a severe physiological disorder, common to many fruit crops. It affects fruit quality in numerous species of fleshy fruits (Brüggenwirth and Knoche, 2017; Butani et al., 2019; Lara et al., 2019; Schumann et al., 2019; Wang et al., 2021b).These include sweet cherry, plum, apricot, apple, litchi, pomegranate, citrus, banana, avocado, grape, persimmon, peach, tomato, and pistachio (Simon, 2006; Khadivi-Khub, 2015; Correia et al., 2018; Butani et al., 2019). However, sweet cherry, grape and tomato are the crops most affected by cracking due to their susceptibility to damage associated to the large scale of these industries (Brüggenwirth and Knoche, 2017; Schumann et al., 2019). Cracks on the fruit surface reduce the fruit marketability as they cause negative effects in fruit quality such as poor appearance, shelf life diminution and increased susceptibility to infections by fungi and other pathogens causing significant losses in the fresh market (Khadivi-Khub, 2015; Butani et al., 2019; Wang et al., 2021b). So, the cracked fruits can only be used in processing industries (especially for fruit juice) if they aren’t infected by fungi (Simon, 2006; Khadivi-Khub, 2015).
In fruits, the skin, also designed as exocarp, supports the internal cell layers, being considered an essential element in fruits which provides a protective barrier against water loss and pathogen attack (Macnee et al., 2020). The fruit skin comprises three main layers, namely cuticle, epidermis and hypodermis (Knoche and Winkler, 2019). Among them, the hypodermis comprises one to several layers of hypodermal cells while the epidermis is located outside the hypodermis, consisting of in just one cell layer. Epidermis and hypodermis comprise the fruit skin (Knoche and Lang, 2017). The arrangement of hypodermal and epidermal cell layers as well as their thickness highly affect the fruit cracking (Wang et al., 2021b). Outside the epidermis, there is a cuticle or cuticular membrane consisting in lipid polymer that covers the fruit surface, acting as a primary barrier for transport of substances into and out of fruits (Weichert and Knoche, 2006). It also plays an important role in the mechanical properties of the skin (Knoche and Lang, 2017). Thus, the exocarp is a key target in many breeding programs related to cracking (Macnee et al., 2020).
Cracking index (CI) refers to the percentage of cracked fruits in the orchard (Correia et al., 2018). Determining the total number of cracked fruits according the orchards conditions is the most reliable method to access the CI (Christensen, 1972). However, this determination depends on climate conditions as well as the fruit stage development or cultivar, which compromises the method accuracy (Christensen, 1972). Considering the sweet cherry, in the lab, CI can be determined as CI= , where a, b and c indicate the number of cracked fruits after 2, 4 and 6h of fruits immersion in distilled water (Christensen, 1972; Correia et al., 2018). Concerning to the position of cracks, there are three main types of fruit cracking: (1) deep cracks in the side of fruits, also called as lateral cracking; (2) small/fine cracks at the fruit apical end, also called as pistillar end and (3) circular or semicircular cracks around the stem end in the cavity region (Simon, 2006; Khadivi-Khub, 2015; Rehman et al., 2015; Correia et al., 2018).
The combination of genetic and environmental factors makes fruit cracking difficult to study, even in controlled conditions. Thus, the basic mechanisms involved in cracking remain unclear. However, researchers have suggested that the high occurrence of fruit cracking can be influenced by several factors, namely physiological, biochemical, environmental, agronomical cultural, anatomical, genetic and postharvest storage factors (Simon, 2006; Khadivi-Khub, 2015; Rehman et al., 2015; Correia et al., 2018; Wang et al., 2021b). Simon (2006) suggested that the species and cultivars susceptibility to cracking is mainly genetic. The two main environmental factors involved in cracking are the quantity of rain and its distribution during the ripening period as well as the soil type (Simon, 2006). Cracking may occur during fruit growth, development and ripening (Khadivi-Khub, 2015). However, it mainly occurs during fruit ripening due to changes in the biochemical properties of the exocarp (Petit et al., 2017; Lara et al., 2019). When fruit tissues are subjected to pressures higher than the mechanical resistance of their cell walls and cuticle, the cracks appear in fruit skin (Brüggenwirth and Knoche, 2017). This mostly occurs when maturation and harvest time coincide with a period of high humidity, causing water movement from the branches and leaves to the fruits due to a large difference in their water potentials (Lara et al., 2014; Petit et al., 2017). Moreover, the combination of high temperatures and low humidity, which make the fruit’s skin hard and inelastic, followed by heavy rains accelerates the growth and expansion of the internal tissues at a faster rate. Once the fruit’s skin remains inelastic and their growth doesn’t coup up with the internal tissues growth, cracks appears in the fruit skin (Butani et al., 2019). This leads to a constant stress supported by the fruit since, in most species, the fruit surface and volume increase during fruit development (Knoche and Lang, 2017). Thus, an uncoordinated internal growth associated to an external environment with high climatic variability results in the appearance of cracks in the fruit surface (Wang et al., 2021b).
Using sweet cherry as a model, cracking is commonly associated adverse environmental conditions. These include rain, wet weather and excessive osmotic water uptake through the fruit surface and skin, fruit peduncle cavity, and also fruit peduncle. Excessive water leads to an increase of flesh turgor, fruit volume and surface. Cracks develop when the limit of extensibility of its skin is exceeded (Weichert and Knoche, 2006; Winkler et al., 2016; Knoche and Lang, 2017). This possible explanation for cracking, known as a critical turgor hypothesis, suggests that fruit peduncle, presence of cracks and cuticle are potential pathways for water uptake in sweet cherry (Knoche and Peschel, 2002; Knoche and Winkler, 2019). Water uptake may occur during and after rainfall when water remains in sweet cherry surface as it is retained in the peduncle cavity and in the stylar end leading to a continuous water uptake after rain (Beyer et al., 2005; Knoche and Winkler, 2019). Another possible explanation for sweet cheery cracking, known as a zipper hypothesis, suggests that a localized skin rupture occurs like a zipper due to a local exposure of skin to water where a succession of events leads to cracks development (Winkler et al., 2016). Strain in the skin during the last stage of fruit growth occurs due to a down regulation of genes involved in wax and cutin biosynthesis, leading to a decrease in cuticle deposition (Alkio et al., 2012; Alkio et al., 2014). A thinner cuticle may not withstand increase of strain in the skin and, consequently, microcracks develop (Winkler et al., 2016).
Fruit cracking in sweet cheery can occur due to several additional factors, because of different cracking susceptibilities of cultivars. These include fruit size and firmness, fruit shape, skin and cuticular properties, osmotic concentration and stomata in the fruit skin, stage of fruit development, and water-retaining capacity of the fruit pulp (Simon, 2006; Balbontín et al., 2013; Khadivi-Khub, 2015; Rehman et al., 2015; Correia et al., 2018). Moreover, Li et al. (2021a) proposed that orchard management like irrigation, growth regulators or mineral applications as well as gene expression related to fruit traits may have a positive relationship with cracking. Simon et al. (2004) reported a positive correlation between cracking and soluble solids content.
Cuticle as an interface fruit-environment
The cuticle is very important in flesh fruits, as it acts as a mechanical protective barrier against external or internal stresses, either biotic or abiotic, and in defense against pathogens (Petit et al., 2017; Lara et al., 2019; Trivedi et al., 2019). The cuticle is composed of a lipophilic polymer of cutin, waxes, comprising a mixture of very-long-chain fatty acids and their derivatives, and polysaccharides (Knoche and Winkler, 2019; Trivedi et al., 2019). It is a primary barrier in water transport and fruit rot pathogens, responding to environmental conditions like water deficit, changes in relative humidity, temperature or light intensity (Knoche and Winkler, 2019; Lara et al., 2019). It also provides mechanical support for fruit integrity (Zarrouk et al., 2018). So, the cuticle weakening in ripe fruits can cause severe economic losses by developing several visual cuticle-associated traits which are dependent of the interaction among the cuticle and environment and/or the development stage of the fruit (Petit et al., 2017; Lara et al., 2019). Among the several visual cuticle-associated traits can be included fruit color in tomato (Gonzali and Perata, 2021), fruit cracking in sweet cherry (Lane et al., 2000; Simon, 2006; Rehman et al., 2015), tomato (Domínguez et al., 2012), pomegranate (Singh et al., 2020), grape (Sahadev et al., 2017) or litchi (Marboh et al., 2017), brightness in tomato (Petit et al., 2014), russeting in apple (Knoche et al., 2011; Straube et al., 2021) and pear (Zhang et al., 2020; Zhang et al., 2022), and browning in pear (Franck et al., 2007) and litchi (Jiang et al., 2004). The thickness and chemical composition of fruit cuticle is another factor in cuticle-associated traits, presenting a high variability according fruit tree species, cultivars, and fruit development (Knoche and Lang, 2017; Zarrouk et al., 2018). Although the cuticle-associated traits have a considerable phenotypic diversity, they can be linked to genotypic variation (Petit et al., 2017). However, to understand the cuticle-associated traits in crop species, it is essential to identify new cuticle-related genes and the alleles involved in the trait-of-interest to select beneficial cuticle-associated genetic variants for genetic improvement (Petit et al., 2017; Lara et al., 2019). Thus, the identification of genes that play a role in cuticle synthesis and deposition is important to obtain a better knowledge of its function and development (Lara et al., 2014; Lara et al., 2019; Wang et al., 2021b). Identifying genes involved in cuticle development may contribute to develop cracking resistant cultivars by maintaining the cuticle barrier function, and, thus prevent microcracks formation, keep low stomatal density and a thick cuticle (Peschel and Knoche, 2012).
Molecular mechanisms associated to cracking
Cracking susceptibility of cultivars is considerable among the different species affected by this disorder. Different fruit cultivars present different cracking phenotypes. It is interesting that a cultivar totally tolerant to the disorder has not been described (Balbontín et al., 2013; Butani et al., 2019), this maybe due to a quantitative gene effect based on multiple genes (Khadivi-Khub, 2015; Wang et al., 2021b). So, understanding the genetic factors involved in fruit cracking is essential to select and develop crack-resistant cultivars, which has been one of the major goals in most of the breeding programs (Balbontín et al., 2013; Khadivi-Khub, 2015).
One aim of sweet cherry breeding strategies is to develop more cracking resistant cultivars. Resistance may be associated with genotypes that present low cuticle strain and thick cuticle, maintaining an intact cuticle throughout fruit development. A second type may be genotypes that maintain cutin and wax deposition along fruit growth, especially in the last stage of fruit development (Peschel and Knoche, 2012) Indeed, the characterization of genes related to fruit cuticle development can provide more knowledge about the cuticle functions (Lara et al., 2014). Transcriptomic analyses, shows changes in the expression level of some genes, potentially involved in wax biosynthesis, consistent with wax concentrations (Lara et al., 2019). These include genes related to waxes and cutin biosynthesis and cuticular lipid transporters, whose downregulation leads to a cessation of cuticle deposition (Alkio et al., 2012; Alkio et al., 2014). The cuticular waxes composition varies among fruit species and cultivars (Trivedi et al., 2019).The major plant cuticular waxes components are derived from very-long-chain fatty acids (VLCFAs) and their derivatives like primary and secondary alcohols, alkanes, aldehydes, ketones, and esters (Samuels et al., 2008; Zarrouk et al., 2018; Trivedi et al., 2019). These biomolecules are generated by the de novo fatty acid biosynthesis in the plastid followed by fatty acid elongation in the endoplasmic reticulum of the epidermal cells (Zarrouk et al., 2018).
Genes involved in cell wall metabolism affect fruit cracking (Wang et al., 2021b). Cracking rate is influenced by cell wall protopectin and cellulose contents and cell wall thickness (Jiang et al., 2019a). Moreover, the mechanical characteristics of the pericarp, determined by cell wall disassembly, modification, and composition can also contribute to cracking susceptibility (Brüggenwirth and Knoche, 2017). Plant cell wall metabolism regulates the cell wall extensibility, determining cell size and shape (Le Gall et al., 2015). Cell wall degradation and modification has been linked to fruit ripening and softening (Teh et al., 2014). Cell wall-modifying enzymes designated as non-pectolytic enzymes are involved in cell enlargement and expansion by hemicellulose modifications. These include endo-1,4-β-glucanases (EGase), xyloglucan endotransglycosylase/hydrolases (XET/XTH) and expansins (Goulao and Oliveira, 2008; Le Gall et al., 2015). Other cell wall-modifying enzymes, including polygalacturonases (PG), pectin methylesterases (PME), pectin acetylesterases (PAE), pectin/pectate lyases (PL) and β-galactosidases (β-Gal), are involved in cell wall plasticity by cleavage or modification of the polysaccharide backbone. Thus, they act as pectolytic enzyme expansins (Goulao and Oliveira, 2008; Le Gall et al., 2015). The properties and structure of cell walls are affected by modifications on the cell wall polysaccharides during ripening (Brummell, 2006). These have been associated to the development of fruit cracking as a result of combined action of cell wall modifying enzymes during fruit ripening and softening (Brummell and Harpster, 2001). Xyloglucan endotransglycosylase is involved in cell wall expansion and re-modelling (Stratilová et al., 2020) by hydrolyzing and re-ligating xyloglucan to other polysaccharides, especially with cellulose. It may control the cell wall relaxation, as the interaction among xyloglucan and cellulose affects plant cells growth control and fruit softening (Kaur, 2019).
Plant growth, both in cell size and number drive fruit expansion and must overcome resistance from the protective cell wall (Marowa et al., 2016). The expansins are involved in cell wall extension acting as regulators of plant cell elongation. Expansins contribute to fruit ripening and softening (Li et al., 2003; Marowa et al., 2016). Expansins, act as zippers to break the hydrogen bonds and unlink cell wall polysaccharides (Marowa et al., 2016). Expansins have been associated with a decrease in cracking index, as they promote the fruit growth by cell walls extensibility and cell expansion (Marowa et al., 2016).
Plant cell wall-modifying enzymes play a key role in fruit ripening, being encoded by multigene families, highlighting their complexity (Brummell, 2006; Goulao and Oliveira, 2008). Moreover, there are increases in expression as well as de novo synthesis and activity of cell wall-modifying enzymes, promoting significant modifications in cell wall during ripening (Goulao and Oliveira, 2008).
Transcription factors (TFs) regulate gene expression acting as molecular switches of their target genes binding to different cis-regulatory elements (Franco-Zorrilla et al., 2014; Joshi et al., 2016; Javed et al., 2020). They control all developmental aspects in living cells (Javed et al., 2020). TFs present an important role in plant tolerance/resistance to both biotic and abiotic stresses (Shahzad et al., 2021), by suppressing or activating genes at the transcriptional level (Javed et al., 2020). Some TFs are crucial in biotic and abiotic stresses simultaneously, and also a single TF has the capacity to answer to several stresses (Shahzad et al., 2021). In plants, there are more than 50 TFs families, being WRKY, MYB, NAC (Javed et al., 2020; Shahzad et al., 2021), AP2/ERF (Javed et al., 2020), DREB, bZIP, Zinc-finger, HSF, Dof and NF-Y (Shahzad et al., 2021) the most important involved in biotic and abiotic stresses. So, a better knowledge about TF genes expressed under multiple stresses, may be useful in new crop breeding programs to develop climate-resilient cultivars as well as improve plants yield and health, since an upregulation of TFs is closely related to an increase of tolerance against biotic and abiotic stresses (Shahzad et al., 2021).
In this context, the review will focus on the main cuticle and cell wall related genes potentially involved in fruit cracking (Figure 1). The potentially cracking-related genes in the several fruits highly affected by cracking, such as sweet cherry (Prunus avium), apple (Malus domestica), watermelon (Citrullus lanatus), litchi (Litchi chinensis), tomato (Solanum lycopersicum), atemoya (A. cherimola× A. squamosa), grape (Vitis vinifera) and jujube (Zizyphus jujuba), are summarized in Tables 1–6 according their putatively role, namely cuticular membrane and cell wall metabolisms (Table 1), cutin biosynthesis and deposition (Table 2), cuticular waxes biosynthesis (Table 3), water transport, calcium transport and signaling, and starch and sucrose metabolism (Table 4), fruit hormone metabolisms (Table 5) and transcription factors (Table 6).
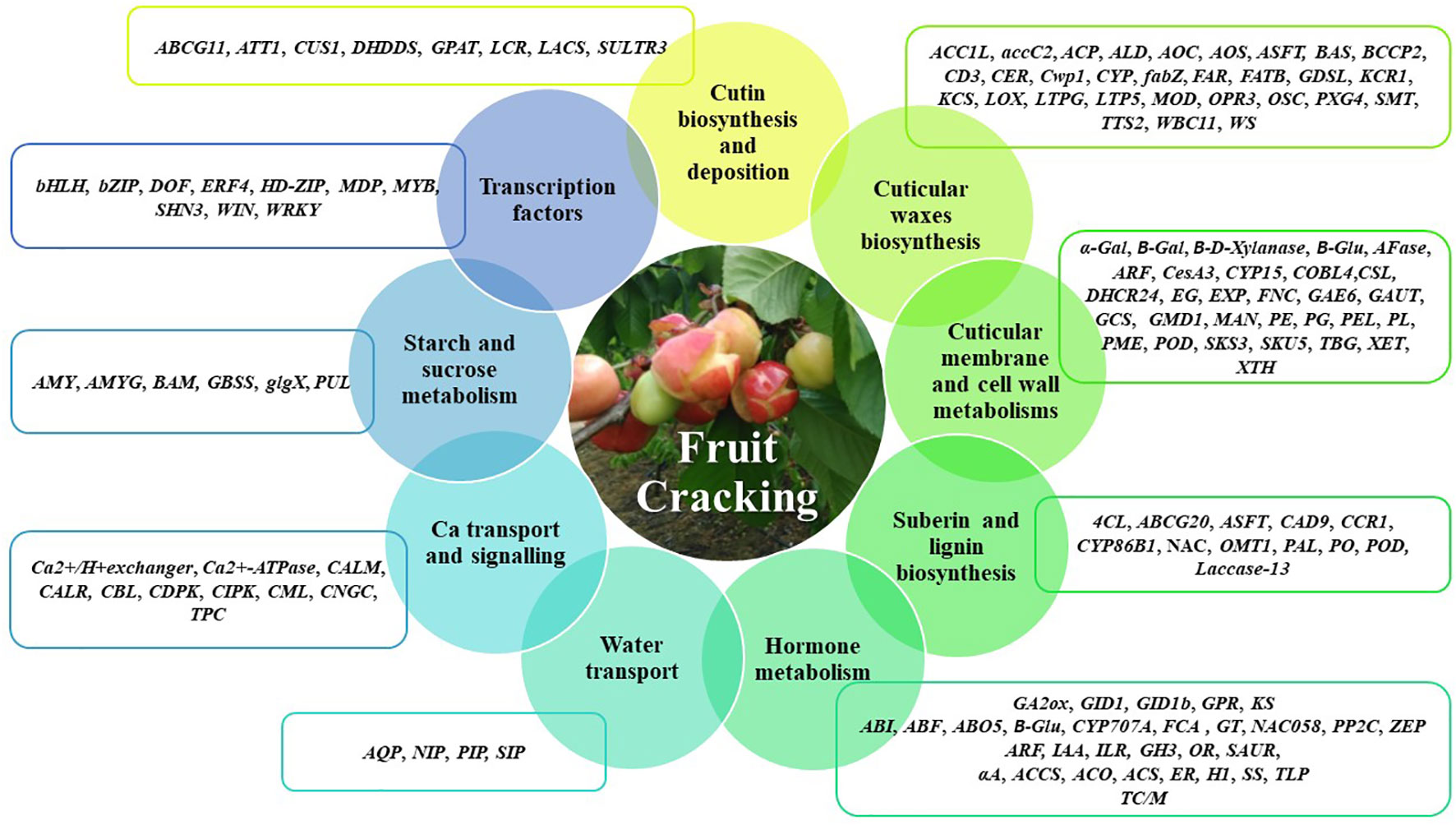
Figure 1 Main gene classes potentially involved in fruit cracking. The genes described belong to the molecular functions of cutin biosynthesis and deposition, cuticular waxes biosynthesis, cuticular membrane and cell wall metabolisms, suberin and lignin biosynthesis, hormone metabolism, water transport, Ca transport and signaling, starch and sucrose metabolisms and transcription factors. Cutin biosynthesis and deposition - ABCG11 (ATP binding cassette transporter), ATT1 (Cytochrome P450 oxidase CYP86A2), CUS1 (cutin synthase 1), DHDDS (ditrans,polycis-polyprenyl diphosphate synthase), GPAT (Glycerol-3-phosphate acyltransferase), LCR (Cytochrome P450 oxidase CYP86A8), LACS (Long chain fatty acid–CoA synthetase), SULTR3 (sulfate transporter 3); Cuticular waxes biosynthesis - ACC1L (acetyl-CoA carboxylase 1-like), accC2 (biotin carboxylase 2, chloroplastic), ACP (Acyl carrier protein), ALD (aminotransferase ALD, chloroplastic-like), AOC (oxide cyclase), AOS (allene oxide synthase), ASFT (Aliphatic suberin feruloyl-transferase), BAS (Beta-amyrin synthase), BCCP2 (biotin carboxyl carrier protein of acetyl-CoA carboxylase 2, chloroplastic), CD3 (cutin deficient 3), CER (Eceriferum family), Cwp1 (cuticular water permeability), CYP (cytochrome-P450 family), fabZ (3-hydroxyacyl-[acyl-carrier-protein] dehydratase FabZ-like), FAR (fatty acyl reductase), FATB (Fatty acyl–ACP-thioesterase B), GDSL (GDSL lípase), KCR1 (b-Ketoacyl-CoA reductase 1), KCS (β-ketoacyl-CoA synthase), LOX (lipoxygenase), LTPG (glycosylphosphatidylinositol-anchored lipid protein), LTP5 (lipid transfer protein 5), MOD (Microsomal oleate desnaturase), OPR3 (12-oxophytodienoate reductase 3), OSC (oxidosqualene cyclase), PXG4 (Putative peroxygenase 4), SMT (24-methylenesterol C-methyltransferase), TTS2 (triterpene synthase 2), WBC11 (ABC-transporter WBC11), WS (wax synthase); Cuticular membrane and cell wall metabolisms - α-Gal (α- galactosidase), B-Gal (β-galactosidase), B-Glu (β-glucosidase), AFase (alpha-L-arabinofuranosidase), ARF (arabinofuranosidase), CesA3 (cellulose synthase), CYP15 (cytochrome P450 monooxygenase/hydrolase), COBL4 (COBRA-like gene 4), CSL (Cellulose synthase-like), DHCR24 (Delta24-sterol reductase), EG (endoglucanase), EXP (Expansin), FNC (Fruit netted-cracking gene), GAE6 (UDP-glucuronate 4-epimerase), GAUT (alpha-1,4-galacturonosyltransferase), GCS (Gamma-glutamylcysteine synthetase, gamma-GCS), GMD1 (GDP-mannose 4,6-dehydratase 1), MAN (Beta-mannanendohydrolase), PE (pectinesterase), PG (polygalacturonase), PEL/PL (pectate lyase), PME (pectin methylesterase), POD (Peroxidase), SKS3 (Pectinesterase-like), SKU5 (Pectinesterase-like), TBG (tomato β-galactosidase), XET (xyloglucan:xyloglucosyl transferase), XTH (Xyloglucan endotransglycosylase/hydrolase); Suberin and lignin biosynthesis - 4CL (4-coumaric acid, CoA ligase), ABCG20 (ATP-Binding cassette G20), ASFT (aliphatic suberin feruloyl-transferase), CAD9 (cinnamyl alcohol dehydrogenase9), CCR1 (cinnamoyl CoA reductase), CYP86B1 (Cytochrome P450), NAC (NAC domain containing protein), OMT1 (O-methyltransferase1), PAL (phenylalanine ammonia lyase), PO/POD (Peroxidase); Hormone metabolism - GA2ox (Gibberellin 2-oxidase), GID1 (GA insensitive DWARF1), GPR (Gibberellin-regulated protein), KS (ent-kaurene synthase), ABI (ABA insensitive), ABF (ABRE-binding factors), ABO5 (ABA overly-sensitive 5), B-Glu (β-glucosidase), CYP707A (ABA 8’-hydroxylase), FCA (Flowering time control protein A), GT (ABA glycosyltransferase), NAC058 (ABA signaling gene - NAC Domain containing protein58), PP2C (Protein phosphatase 2C), ZEP (Zeaxanthin epoxidase), ARF (Auxin response Factor), IAA (indole-3-acetic acid), ILR (IAA-amino acid hydrolase), GH3 (IAA-amido synthetase), OR (Oxidoreductase) (NAD+ oxidoreductase), SAUR (Small Auxin Up-Regulated genes), αA (alpha-amylase), ACCS (Aminocyclopropanecarboxylate synthase), ACO (1-aminocyclopropane-1-carboxylic acid oxidase), ACS (1-aminocyclopropane-1-carboxylic acid synthase), ER (Ethylene receptor), H1 (Hydrolase), SS (sucrose synthase), TLP (Thaumatin-like protein), TC/M (terpene cyclase/mutase); Water transport - AQP (Aquaporin), NIP (Aquaporin), PIP (Plasma membrane intrinsic protein), SIP (Aquaporin); Ca transport and signalling - CALM (Calmodulin), CALR (Calreticulin), CBL (calcineurin B-like protein), CDPK (Ca2+-dependent protein kinases), CIPK (CBL-interacting serine/threonine-protein kinase), CML (Calmodulin-like protein), CNGC (Cyclic nucleotide-gated ion Channel), TPC (putative voltage-gated Ca2+); Starch and sucrose metabolism - AMY (alpha-amylase), AMYG (glucoamylase), BAM (beta-amylase), GBSS (granule-bound starch synthase), glgX (glycogen debranching enzyme), PUL (pullulanase); Transcription factors - bHLH (bHLH transcription factor), bZYP (bZIP transcription factor), DOF (DOF transcription factor), ERF4 (ethylene-responsive transcription factor 4), HD-ZIP (homeobox-leucine zipper protein), MDP (MADS box transcription factor), MYB (MYB domain protein), SHN3 (ethylene response subfamily member), WIN (AP2/EREBP-type transcription factor), WRKY (WRKY family transcription factor).
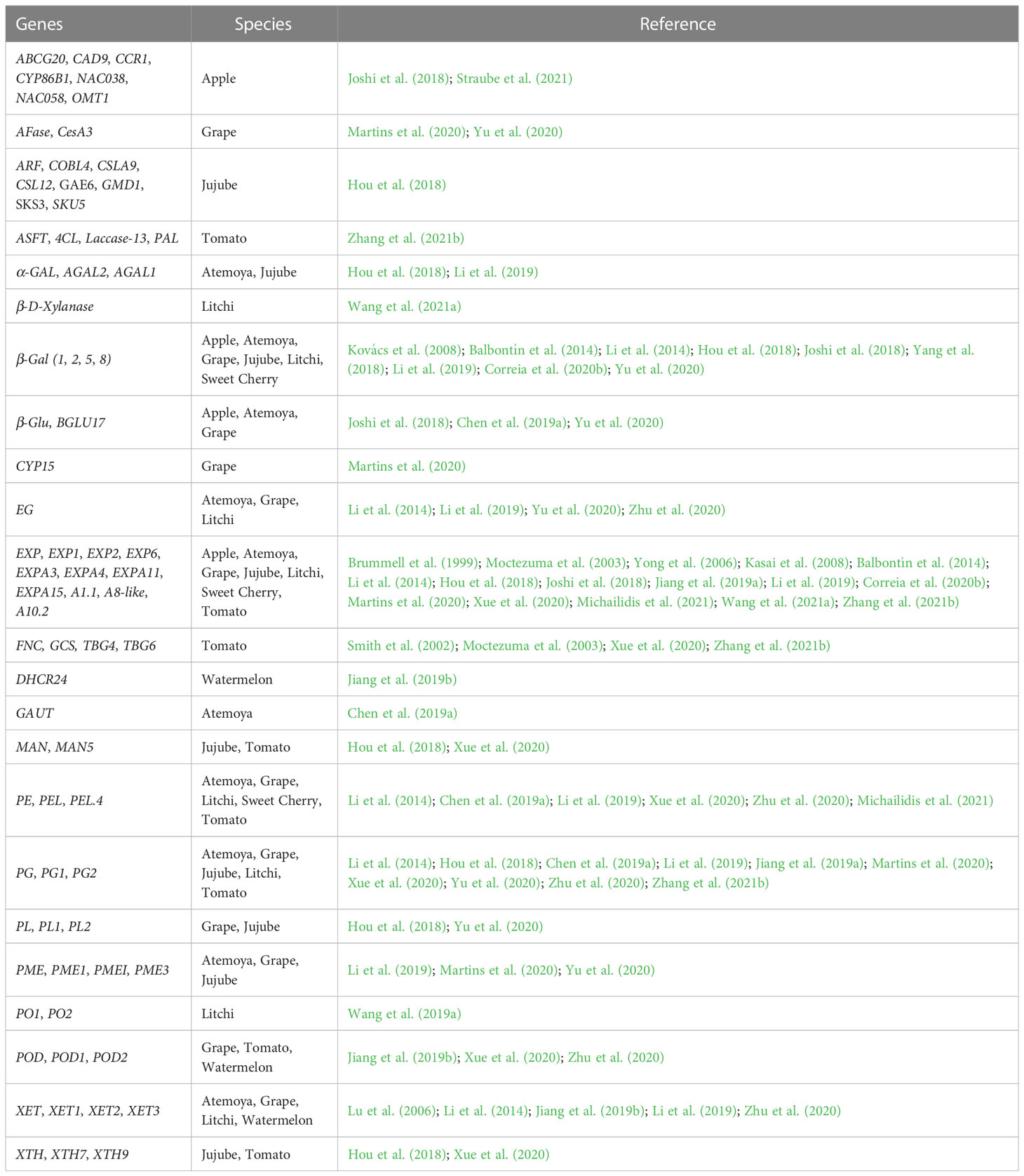
Table 1 Potentially cracking-related genes involved in metabolisms of cuticular membrane, cell wall, suberin and lignin biosynthesis.
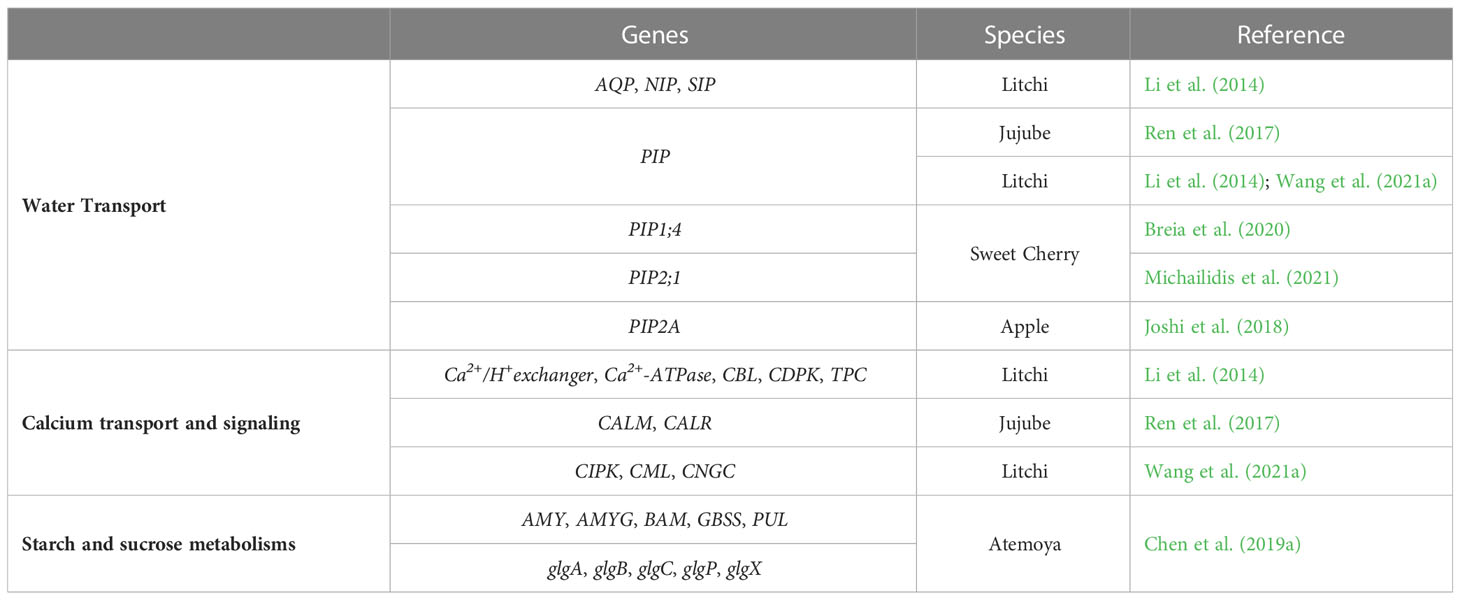
Table 4 Potentially cracking-related genes involved in water transport, calcium transport and signaling, and starch and sucrose metabolisms.
Cracking-related genes involved in cuticular membrane, cell wall, suberin and lignin biosynthesis
The first work about genes involved in the cuticle formation in sweet cherry was published by Alkio et al. (2012). Based on sequence similarity with Arabidopsis, they used the cultivar Regina to identify genes potentially relevant for cuticular membrane (CM) formation. Among the 18 CM target genes identified by Alkio et al. (2012), 15 of them were only detected in the exocarp, meaning that these genes are exocarp-specific. Moreover, 13 of the exocarp-specific genes present a positive correlation with CM deposition, that is, their transcription levels are high when the CM deposition rate is high and low when CM deposition is low. Generally, these genes have higher expression during the first stage of fruit development, when CM deposition is high (Alkio et al., 2012). In sweet cherry, the maximum β-galactosidase activity occurs in the early stages of active growth and then decrease abruptly during ripening (Kovács et al., 2008). Similarly, the results provided by Balbontín et al. (2014) also refer that transcript levels of β-galactosidase gene vary during fruit development, showing their highest transcript levels in the fruit set stage, declining as ripening advances (Table 1). Likewise, Correia et al. (2020b) found different expression levels during fruit development and under different applied compounds, like gibberellic acid, salicylic acid or calcium, in Sweetheart, a cultivar with moderate resistance to cracking. The expansin, EXP1, has higher expression in the ripening stage in Kordia, a cracking-resistant cultivar, while in Bing, a cracking-susceptible cultivar, has higher expression in the fruit setting and fruit color change stage (Balbontín et al., 2014). This data has been confirmed by Correia et al. (2020b). There is an increase in expression levels of EXP1 during fruit development in a cracking-moderate resistant cultivar Sweetheart. Moreover, the expression of the most abundant expansins in sweet cherry (A1.1, A8-like, A10.2) are upregulated in a moderately resistant cultivar Regina compared to the cracking-susceptible cultivar Early Bigi (Table 1) (Michailidis et al., 2021). These findings are in agreement with Balbontín et al. (2014) when attested that the more cracking-resistant cultivar present higher gene expression in all stages as well as Correia et al. (2020b) who verified that cherries treated with biostimulant (Ascophyllum nodosum) and growth regulators (eg. abscisic acid, glycine betaine or salicylic acid) have lower cracking index, presenting higher transcripts levels of the studied genes, which increase their expression during fruit development. The gene related to pectin metabolism, PEL.4, has higher expression levels in the skin of a cracking-susceptible cultivar Early Bigi (Michailidis et al., 2021).
Expansins also play a role in apple fruit development. Wakasa et al. (2003) identified six expansin genes and studied their expression patterns during fruit growth, being EXPA3 mainly expressed during the fruit enlargement phase. The same was later described by Joshi et al. (2018) for EXPA4 gene. Moreover, EXPA3 transcripts in the mesocarp are higher at the fruit color change stage. In the pericarp, EXPA3 expression is higher at the begin of fruit development and in the ripening stage (Wakasa et al., 2003). This indicates that an accumulation of EXPA3 mRNA in pericarp reduces the susceptibility of fruit cracking. Early symptoms of fruit cracking coincide with situations in which EXPA3 gene expression in the mesocarp exceeds the expression in the pericarp (Table 1) (Kasai et al., 2008). Expression levels of β-Gal genes increase during apple fruit growth and are higher in the mature fruits of cultivar Fuji, a softer and crisper apple, than in fruits of cultivar Qinguan, a firmer and tougher apple (Yang et al., 2018). Among them, β-Gal1, β-Gal2, and β-Gal5 genes are highly expressed in fruits, presenting a significant increase of expression patterns until fruit ripening, which suggest that these genes can affect the fruit texture in both types of apple cultivars (Yang et al., 2018). Similarly, there is an upregulation during apples development for BGAL8 and BGLU17 cell wall related genes (Table 1) (Joshi et al., 2018). Furthermore, an upregulation of genes involved in suberin and lignin synthesis, namely ABCG20, CYP86B1, NAC038 and NAC058, leads to an increase in suberin content and periderm formation, and thus, to the microcracks development and russet apples (Table 1) (Straube et al., 2021). In contrast, an upregulation of the lignin-biosynthesis genes, CAD9, CCR1 and OMT1 can prevent crack initiation (Table 1) (Joshi et al., 2018).
Regarding to suberin and lignin related genes in watermelon, POD1 gene is upregulated in the cracking-resistant watermelon, while POD2 is downregulated in cracking-susceptible watermelon. Similarly, the genes involved in cell wall mechanisms, XET1, XET2 and DHCR24 (Table 1) are downregulated in cracking-susceptible watermelon (Jiang et al., 2019b).
Concerning to cell wall related genes in litchi, the analysis of XET1, XET2 and XET3 genes has different expression patterns among a cracking-resistant cultivar Huaizhi and a cracking-susceptible cultivar Nuomici, but only XET1 is fruit-specific, once XET1 transcripts accumulation appeared in pericarp while XET2 and XET3 transcripts accumulation enhanced in aril tissues, suggesting that they may play different roles in litchi aril and pericarp growth, and thus, XET1 is more likely to play a role in reducing litchi fruit cracking than XET2 and XET3 (Table 1) (Lu et al., 2006). Additionally, the expression of a XET gene is upregulated in fruits without cracks compared to cracked fruits (Li et al., 2014). The expression of two genes encoding expansins in litchi pericarp, Exp1 and Exp2, appear to have a closely association with fruit growth and cracking, since the expression of both genes is detected from the early stage of fruit rapid growth and then increase and reach to the highest level at the end of the growth phase in pericarp of the cracking-resistant cultivar Huaizhi, while Exp1 gene is detected at the stage of rapid fruit growth, and then increase slightly and finally kept almost constant in pericarp of the cracking-susceptible cultivar Nuomici, not being detected expression of Exp2 in this cultivar (Yong et al., 2006). Similar results were obtained by Wang et al. (2021a), whose an upregulation of cell wall related genes (EXP and β-D-Xylanase) leads to a low mechanical strength and by Li et al. (2014), who found an upregulation of five EXP in fruits without cracks compared to cracked fruits. The same is observed on nine β-Gal genes, which are upregulated in fruits without cracks compared to cracked fruits, while five PG, one EG and three PE genes are upregulated in cracked fruits compared to fruits without cracks (Table 1) (Li et al., 2014). Regarding to suberin and lignin related genes, an upregulation of PO1 and PO2 genes leads to an increase of lignin biosynthesis, resulting in differences in cuticle structure of litchi fruits pericarps with different susceptibilities to cracking compared to cracked pericarps (Wang et al., 2019a).
Bargel and Neinhuis (2005) studied the biomechanics of tomato fruit skin and isolated cuticles, from three cultivars differing in cracking susceptibility and fruit shape, concluding that the cuticle is a mechanically important component of the tomato fruit. Other important contribution to understand the multiple metabolic and genetic phenomena that occur in the fruit skin during ripening was made in tomato by. Mintz-Oron et al. (2008), which describes the differential gene expression at different fruit developmental stages and in different tissues of the fruit and allowed to identify genes expressed specifically in the skin at ripening, such as genes involved in cell wall modification. Also in tomato, a higher expression of FCN gene also increase the expression of other genes involved in different metabolic pathways such as suberin metabolism genes (ASFT and GPAT5), lignin metabolism genes (4CL, PAL and Laccase-13), and cell wall metabolism genes (EXPA11 and PG), which affects the cell wall extensibility, fruit softening, pericarp firmness leading to the appearance of cracks in all fruit surface (Table 1) (Zhang et al., 2021b). The simultaneous suppression of PG and EXP1 in ripening fruits reduces cell wall disassembly since pg/exp fruits are more firm, present more protopectin and thicker cell walls, concluding that a ripe fruit with more intact pectins in its primary walls is more resistant to cracking (Jiang et al., 2019a). Likewise, antisense inhibition of PE and PG activity affects the level of fruit cracking, while suppression of the ripening related expansin gene (Exp1) (Brummell et al., 1999) and tomato β-galactosidase 4 (TBG4) (Smith et al., 2002) increases fruit firmness. On the other hand, the relationship between activity of cell wall enzymes and cuticular layer was demonstrated in tomato by Moctezuma et al. (2003) as a result of antisense suppression of a β-galactosidase gene (TBG6), observing a positive correlation between cracked fruit number with low levels of β-galactosidase transcripts. The results suggest that the TBG6 product may have an important function in cell wall galactosyl residue metabolism during cell elongation, so the various altered phenotypes observed as a result of TBG6 gene down-regulation in tomato fruit are further evidence that β-galactosidases have important functions in the overall growth and development of tomato fruit (Table 1) (Moctezuma et al., 2003). Additionally, the main genes involved in tomato cracking are associated with different metabolic pathways such as cell wall organization, oxidoreductase activity and catalytic activity, which included genes as MAN, PE, POD, EXP, XTH7, XTH9, PG2, ER, ERF4 and gamma-GCS (Xue et al., 2020). The genes involved in cell wall loosening and expansion, namely XTH7, XTH9, PE and POD, are downregulated in a cracking-resistant cultivar and upregulated in a cracking-susceptible cultivar. Likewise, cell-wall degrading enzyme-associated genes are also upregulated, namely GCS, MAN and PG genes as well as ethylene and auxin responsive genes such as PG, PE, EXP and XTH7 which can be related to cell wall regulation once ethylene influences fruit development and ripening (Xue et al., 2020).
In atemoya, several genes related to cell wall mechanisms were identified, namely 34 PGs, 21 PEs, 19 EXPs, 17 β-GALs, 13 EGs, 6 α-GALs, 6 PME, 4 PELs, 4 XETs and 3 cellulases, which, in general, present higher expression levels in cracked fruits than fruits without cracks, that leads to a reduction in skin elasticity and, consequently, fruits cracking (Li et al., 2019). In addition, GAUT gene, involved in pectin synthesis, is upregulated as well as the genes involved in pectin degradation, namely PE, PG and PEL. Likewise, β-glucosidase gene, responsible for cellulose degradation, also is upregulated (Table 1) (Chen et al., 2019a).
The effect of calcium in genes involved in cell modifications was studied by Yu et al. (2020) using a cracking-susceptible cultivar Xiangfei, whose expression was analyzed in grape berry skins after 1, 2 and 3 weeks of the calcium applications comparing to fruits without calcium application (as a control). Among then, five polygalacturonases (PG) and three endoglucanases (EG) were studied, showing a downregulation of almost all PG genes by calcium at all weeks as well as a downregulation of two EG genes in the first and second weeks, and then an upregulation of these genes in the third week (Table 1) (Yu et al., 2020). Similar results were obtained by Zhu et al. (2020) using grapes of the same cultivar, Xiangfei, during the ripening stage, finding an upregulation of two EGs and one PG along the fruit maturation. Other genes involved in cell modifications namely one pectin methylesterase (PME1), two polygalacturonases (PG1 and PG2), one expansin (EXP6) and one cellulose synthase (CesA3) as well as a cuticle biosynthesis gene, cytochrome P450 monooxygenase/hydrolase (CYP15), were studied at pulp and skin of grape berry, also with and without calcium treatment in the cultivar Vinhão, showing a downregulation of the genes PME1, PG1, PG2, EXP6 and CYP15 promoted by calcium in skin and pulp, while CesA3 gene is not significantly affected by calcium in the skin and pulp (Table 1) (Martins et al., 2020). This suggest a regulation by calcium at transcriptional level and also that calcium can inhibit additional enzymatic pathways involved in cell wall mechanisms (Martins et al., 2020). The work performed by Yu et al. (2020) also analyzed four pectate lyases (PL), ten pectin methylesterase (PME), two PME inhibitors (PMEI), one alpha-L-arabinofuranosidase (AFase), four β-galactosidase (β-Gal), and one β-glucosidase (β-Glu), all involved in cell wall modifications, in grape berry skins after 1, 2 and 3 weeks of the calcium applications. Among the analyzed genes, the AFase, the β-Glu, almost all β-Gal genes and one PME are downregulated by calcium at all weeks, while two PME genes are downregulated in the first week after calcium application, four PME genes are downregulated in the second week and three PME genes are downregulated in the third week (Yu et al., 2020). Regarding PL genes, two are downregulated by calcium at first and second weeks and upregulated at third week, while the other two PL genes are upregulated during the three weeks. Moreover, there is also one PMEI gene continuously and significantly upregulated by calcium and another PMEI gene downregulated in the first week (Table 1) (Yu et al., 2020). Based in the results, calcium applications in grape berry appear to induce specific modifications both in skin and in pulp, inhibiting pectin degradation and cell wall loosening, and changing the cuticle structure (Martins et al., 2020) as well as leading to cell wall disassembly inhibition, and promoting cell wall strengthening (Yu et al., 2020), playing an important role in preventing cracking. In order to analyze the transcriptome and identify important metabolisms related to grape berry cracking, Zhu et al. (2020) made a RNA-Seq analysis to assess the expression of pericarp genes during the ripening stage, namely at 1 (W1), 2 (W2) and 3 (W3) weeks after veraison in cultivar Xiangfei. The three groups (W1, W2 and W3) presented a similar number of expressed genes, however with different expression in each group (some genes express in all groups, but some genes only express in one unique group) (Zhang et al., 2021a). Comparing W1, W2 and W3, the authors detected an increase of cracking during repining highlighting great changes in gene expression during this period, which can be correlated with 303 DEGs up-regulated and 354 DEGs down-regulated in W2 and W3 relatively to W1 (Zhu et al., 2020). During the fruit development, the cell wall mechanical properties have an important role (Brüggenwirth and Knoche, 2017), so Zhu et al. (2020) validated the RNA-Seq results with a qRT-PCR analysis of genes involved in cell mechanisms, obtaining highly consistent results for both analysis. Among the DEGs involved in cell wall mechanisms, one peroxidase (POD), one pectinesterase (PE) and eleven xyloglucan endotransglycosylase (XET), beyond two EGs and one PG, were identified (Table 1). These genes have differential expression when W2 and W3 groups are compared to W1, showing an up-regulated expression along fruit maturation, which suggested that cell wall related genes play important roles in grape berry cracking regulation (Zhu et al., 2020).
By a transcriptomic analysis of jujube using young and mature fruit, Hou et al. (2018) found several differentially expressed genes, 19 of them related to cell wall mechanisms and fruit ripening. The analysis of gene expression revealed that AGAL2, BGAL, EXP2, EXPA15, PL2, SKU5, GAE6 and XTH genes are upregulated in young fruit stage while ARF, CSL12, CSLA9, COBL4, GMD1, PL1 and PG1 genes are upregulated in ripe fruit stage. However, AGAL1, MAN5, PME3 and SKS3 genes, don’t present significant expression differences among the two ripening fruit stages (Table 1) (Hou et al., 2018). Thus, these differences in gene expression during fruit development leads to important changes in cell wall metabolisms playing important roles in preventing or enhancing jujube cracking (Hou et al., 2018).
Cracking-related genes involved in cutin biosynthesis and deposition, and in cuticular waxes biosynthesis
In sweet cherry, the expression of genes involved in cutin biosynthesis and deposition, namely LCR and LACS1 genes, is detected in the beginning of fruit development, while expression of ATT1 and LACS2 (Table 2) have higher expression at later fruit stages (Alkio et al., 2012). The previous data was confirmed by Declercq et al. (2014) for LACS2 and ATT1 genes, concluding that the expression of these genes increase cutin deposition and decrease cuticle permeability. They may be involved in the molecular mechanisms of sweet cherry cracking. Additionally, expression of GPAT4/8 is detected in the beginning of fruit development and increase again in the final phase of fruit development (Alkio et al., 2012). Concerning cuticular waxes related genes, more specifically involved in biosynthesis of VLCFAS, KCS6 and KCS1 present higher expression in the beginning of fruit development and increased again in the final phase of fruit development as well as the expression of Lipase (Table 3) (Alkio et al., 2012). Likewise, Balbontín et al. (2014) found higher expression of KCS6 at fruit setting (begin of fruit development) and fruit color change stage in a cracking-susceptible cultivar Bing, and higher expression in a cracking-resistant cultivar Kordia, coinciding with the ripening of fruits. Additionally, the wax synthase gene, WS, presents similar expression levels to KCS6 (Balbontín et al., 2014). These results have been again corroborated by Correia et al. (2020b) analyzing the expression patterns of WS gene in a cracking-moderate resistant cultivar Sweetheart. WS increases during fruit development, leading to higher wax content (Correia et al., 2020b). The genes involved in lipid transport, LTPG1, WBC11 and CER1 show higher expression in the begin of fruit development and, again, in the fruit ripening stage (Table 3) (Alkio et al., 2012). The expression of KCR1 and FATB genes is higher in the exocarp in the beginning of fruit development and again in the final fruit development phase. The expression of CER5 and CER3 genes is also exocarp-specific at all developmental stages but do not correlate with the CM deposition rate (Alkio et al., 2012).
In apples, the presence of microcracks can lead to russeting (rough and brownish patches on the fruit skin) which can occur due to water uptake on the fruit’s surface (Khadivi-Khub, 2015; Straube et al., 2021). Straube et al. (2021) studied the effect of moisture exposure in apples verifying a decrease in cutin and wax contents due to a down-regulation of genes involved in cutin and wax synthesis (ABCG11, GPAT6, KCS10, WSD1 and CER6 genes) (Table 2 and Table 3). This leads to a decrease in cuticle formation and, consequently, to microcracks formation. The LTPG genes codify proteins involved in lipid transport. Studies performed by Joshi et al. (2018) show a significant upregulation of LTPG5 gene as well as of CER3 and ASFT during apple fruit development. The expression of LTPG genes is responsive to abiotic stresses and stress hormones such as drought, cold, salt, salicylic acid and jasmonate (Gao et al., 2021). Among 26 potential LTPG genes, 9 of them are highly expressed in fruits, including LTPG2, LTPG3, LTPG5, LTPG6, LTPG7, LTPG8, LTPG11, LTPG15, and LTPG19, highlighting the function of this gene family in wax biosynthesis and cracking prevention (Table 3) (Gao et al., 2021). Triterpenes are components of surface waxes (Thimmappa et al., 2014). There is a close relationship between the expression of oxidosqualene cyclase (OSC) genes and russeting level in apples. OSC1 and OSC3 genes present low expression in cultivar Rugiada that generally shows fully russeted skin. In contrast, OSC1 and OSC3 are highly expressed in cultivars Smoothee and Golden Delicious with low and moderate russeting, respectively (Falginella et al., 2021). OSC4 and OSC5 genes are downregulated in cultivars Smoothee and Golden Delicious and upregulated in Rugiada, correlating with high russeting level in this cultivar (Table 3) (Falginella et al., 2021). Likewise, the β-amyrin biosynthesis-related genes, BAS and CYP716A1, are upregulated during fruit development (Joshi et al., 2018).
The watermelon rind has an important role in fruit cracking, being the rind hardness positively correlated with cracking resistance of this fruit (Liao et al., 2020). The expression of genes related to cracking was studied in watermelon (Citrullus lanatus), namely cutin related genes, GPAT, DHDDS and SULTR3 (Table 2) and genes involved in cuticular waxes biosynthesis, SMT and KCS (Table 3), using a cracking-resistant and a cracking-susceptible watermelon cultivars (Jiang et al., 2019b). The results show that the DHDDS and SULTR3 genes are upregulated in the cracking-resistant watermelon, while GPAT, SMT and KCS genes are downregulated in cracking-susceptible watermelon (Jiang et al., 2019b).
In litchi (Litchi chinensis), the pericarps of fruits without cracks from cultivars with different cracking susceptibilities and cracked fruits present differences in cuticle structure as a result of an upregulation of genes related to fatty acids such as LOX, MOD and AOS (Table 3) (Wang et al., 2019a) as well as a downregulation of lipid synthesis genes, like ACP, which is responsible by a low pericarp mechanical strength (Wang et al., 2021a). Moreover, the upregulation of AOS gene is found in cracked fruits (Wang et al., 2019b).
The expression of genes related to cutin in tomato, like LACS1 (long-chain acyl-CoA synthase 1), CUS1 (cutin synthase) and GPAT4 (glycerol-3-phosphate acyltransferase 4), decreases during the fruit development and is higher in fruits under water stress, being consistent with the developmental regulation of cuticle (Table 2) (Romero and Rose, 2019). Further evidence of the relationship between fruit cracking and properties and composition of the cuticle in tomato is provided by Vogg et al. (2004), where the mutation of the CER6 gene (β-ketoacyl-CoA synthase) leads to an alteration of the cuticular wax composition and increases water permeability (Hovav et al., 2007). Additionally, the genes TTS2 (triterpene synthase 2) and CD3 (cutin deficient 3) beyond CER6 (eceriferum 6), all involved in wax biosynthesis, transport, deposition and regulation, decrease their expression during the fruit development and are higher in fruits under water stress, suggesting that water availability affects the cuticle properties (Table 3) (Romero and Rose, 2019). Moreover, the Cwp1 gene (cuticular water permeability) when expressed leads to fruit dehydration and consequently causes microcracks in tomato cuticle (Hovav et al., 2007). The higher expression of FNC gene is responsible by cracking all over the tomato pericarp, whose expression affects other cracking related genes, namely by increasing the expression of genes involved in lipid metabolism such as GDSL, KCS and FAR, and lipid transport like NLTP9 and LTP5, affecting the cuticle elasticity and wax content and thud, cracks appear in fruit surface (Table 3) (Zhang et al., 2021b).
Concerning to genes related to wax formation in grape, Martins et al. (2020) verified a downregulation of one E3 ubiquitin ligase (CER9) when calcium was applied in the cultivar Vinhão (Table 3).
In last years, several studies related to biosynthesis of cuticular waxes and related genes in jujube have been developed (Li et al., 2020; Liu et al., 2020; Li et al., 2021b; Li et al., 2021c). A RNA-Seq analysis, using fruits with and without cracking, to analyze the genes differentially expressed in cracked and non-cracked jujube fruits, allowed to find 785 up-regulated and 251 down-regulated genes in cracked fruits, which are involved in several metabolic processes, namely surface wax production in cracked fruits (Liu et al., 2020). The expression of genes involved in fatty acid biosynthesis (FAR2) and fatty acid elongation (KCS1 and KCS12) was studied in jujube fruits at different maturation stages, namely white-ripe, coloring, and full-red development stages, collected from cultivars with different cracking susceptibilities (a highly cracking-resistant cultivar Popozao, a cracking-resistant cultivar Banzao, and a cracking-susceptible cultivar Hupingzao), showing higher expression for FAR2 and KCS12 genes in coloring stage, being higher in the more resistant cultivar, and lower in the more susceptible cultivar while KCS1 gene presents similar expression during fruit development and for all cultivars (Li et al., 2020). Moreover, the 3-ketoacyl-CoA synthase (KCS) gene, related to the synthetic pathway of cutin, is highly upregulated in cracked fruits, which leads to alterations in biosynthesis in cuticle wax and, consequently, jujube cracking (Table 3) (Liu et al., 2020). Furthermore, Li et al. (2020) also analyzed the genes involved in fatty acid degradation, namely ALD1, ALD4 and ALDH3F1 genes, whose expression of ALD1 and ALDH3F1 genes is upregulated in coloring stage in cracking-resistant cultivar while the expression of ALD4 gene is upregulated in cracking-susceptible cultivar at the same stage (Table 3). Likewise, a transcriptomic analysis carried out by Li et al. (2021c) to access the wax metabolism pathways in jujube using RNA from fruit pericarp at different maturation stages of a cracking-resistant cultivar and a cracking-susceptible cultivar allowed to identify different metabolic pathways related to wax metabolism, namely fatty acid biosynthesis, fatty acid metabolism and cutin, suberin and wax biosynthesis. All identified genes, in general, increase their expression during fruit development; however, genes CER1L1 (eceriferum 1-like), BCCP2 (biotin carboxyl carrier protein of acetyl-CoA carboxylase 2, chloroplastic), ACC1L (acetyl-CoA carboxylase 1-like) and CYP86A22 (cytochrome-P450 86A22) have more expression in cracking-resistant cultivar, while genes accC2 (biotin carboxylase 2, chloroplastic) and fabZ (3-hydroxyacyl-[acyl-carrier-protein] dehydratase FabZ-like) present higher expression in cracking-susceptible cultivar (Table 3) (Li et al., 2021c). Concerning to genes involved in jujube wax biosynthesis (CYP94A2, PXG4, CYP86A, CER1 and CYP86B1), in general, are higher in white-ripe period, however PXG4, CYP86A, CER1 and CYP86B1 genes maintain higher expression in the cracking-resistant cultivar than in the other cultivars in coloring stage (Table 3) (Li et al., 2020). CER genes represent a family of genes with an important role in waxes biosynthesis in jujube, being identified 29 candidate genes (named CER1 to CER29), twelve of them present differences in expression among two cultivars with different susceptibilities to cracking (Li et al., 2021b). Among them, CER7, CER14, CER15 and CER16 genes have higher expression in cracking-susceptible cultivar, while CER29 has more expression in cracking-resistant cultivar. In addition, CER26 only has expression in cracking-resistant cultivar (Table 3) (Li et al., 2021b). In the biosynthesis of cuticular waxes, in general, the highly cracking-resistant cultivar present higher gene expression in coloring period while cracking-susceptible cultivars have higher expression in white-ripe, decreasing during fruit development and, thus, during the wax formation, the cracking-resistant cultivar synthesize more very-long-chain alkanes and aldehydes, accompanying the fruit surface enlargement, which reduces the jujube cracking (Li et al., 2020). The biosynthesis of jasmonic acid (JA) associated with α-linolenic metabolism (as the precursor of JA) is the main associated to cracking in which the allene oxide cyclase (AOC), allene oxide synthase (AOS) and 12-oxophytodienoate reductase 3 (OPR3) genes are upregulated, while lipoxygenase 2 (LOX2) gene is downregulated in cracked jujube fruits when compared with non-cracked jujube fruits (Table 3) (Liu et al., 2020).
Cracking-related genes involved in water transport, calcium transport and signaling, and starch and sucrose metabolisms
Aquaporins (AQPs) transport water, and water uptake is associated to rain-induced cracking in sweet cherries. A better knowledge about these proteins in exocarp and the involvement of AQPs in water penetration through microcracks as well as the transcriptional profile of the genes that codify AQPs can provide new finds about sweet cherry cracking (Chen et al., 2019b). Chen et al. (2019b) identified 25 putative aquaporins genes in sweet cherry, 16 of them express in fruit. An example of the aquaporin role in sweet cherry cracking was provided by Michailidis et al. (2021), who verified that the expression of aquaporin gene, PIP2;1, is upregulated in the skin of Early Bigi cultivar (Table 4). Moreover, Breia et al. (2020) found an upregulation of PIP1;4 gene under pre-harvest application of CaCl2 in cultivar Skeena, suggesting that this AQP is involved in water transport and, possibly, in crack prevention. Likewise, the aquaporin gene PIP2A (Table 4) is upregulated during apple development, which may prevent crack initiation (Joshi et al., 2018).
By a high-throughput RNA sequencing (RNA-Seq), the transcriptome of litchi pericarp revealed four genes (AQP, 1; PIP, 1; NIP, 1; SIP, 1) involved in water transport and 13 genes (TPC, 1; Ca2+/H+ exchanger, 3; Ca2+-ATPase, 4; CDPK, 2; CBL, 3) involved in Ca transport (Table 4), whose expression present significant differences among cracked fruits and fruits without cracks (Li et al., 2014). Furthermore, a downregulation of calcium transport and signaling genes, like CIPK, CML and CNGC provoke a decrease of the mechanical strength of pericarp of a cracking-susceptible cultivar Nuomici (Wang et al., 2021a).
The genes involved in starch and sucrose metabolism pathways also appear to have an important role in atemoya cracking, since genes involved in starch synthesis such as glgA, glgB, glgC and GBSS are mainly downregulated in cracked fruits, while genes involved in starch degradation like AMYG, PUL, AMY, BAM, glgX and glgP are mainly upregulated in cracked fruits (Table 4) (Chen et al., 2019a).
By a transcriptomic analysis, a set of 12 cracking-resistant fruits and 12 cracking-susceptible fruits were analyzed to study the gene expression in both types of fruits, finding 218 upregulated genes and 173 downregulated genes, being the aquaporin PIP (involved in water absorption), CALM and CALR (both involved in calcium transport and regulation) genes (Table 4), the most related to jujube cracking (Ren et al., 2017). All these genes have higher expression in cracking-resistant fruits than in cracking-susceptible fruits, playing an important role in preventing cracking (Ren et al., 2017).
Cracking-related genes involved in fruit hormone metabolism
In sweet cherry, the ABF2, ABF3, ABO5, ABI and FCA genes, involved in abscisic acid metabolism, are downregulated in the cracking-susceptible-cultivar Early Bigi, while ACS and ACO genes, involved in ethylene biosynthesis, are upregulated in a cracking-moderate resistant cultivar Regina (Table 5) (Michailidis et al., 2021). As these plant growth regulators are directly involved in abiotic stress signaling they may pose a mechanistic mode of action to understand the environmental effects on fruit cracking in cherry.
During apple fruit development, the ABA signaling gene, NAC058 (Table 5), is upregulated, and may prevent the cracking initiation (Joshi et al., 2018).
In watermelon, CHDH and GST genes (Table 5) are possibly related to hormone metabolism, whose expression of GST gene is upregulated in the cracking-resistant watermelon, while CHDH gene is downregulated in cracking-susceptible watermelon (Jiang et al., 2019b).
A balance among pericarp strength and aril expanding pressure can be responsible by litchi cracking, which can occur due an unbalance of plant hormone metabolisms (Wang et al., 2019b; Wang et al., 2021a). Different expression levels of genes related to hormone metabolism was described by Li et al. (2014), namely in five genes (KS, 2; GA2ox, 2; GID1, 1) involved in GA metabolism and 21 genes (CYP707A, 2; GT, 9; β-Glu, 6; PP2C, 2; ABI1, 1; ABI5, 1) involved in ABA metabolism in fruits with and without cracks (Table 5). Moreover, genes related to auxins (GH3, IAA and ARF), gibberellins (GPRs), and ethylene (ACO and ACS) are downregulated in litchi pericarp, while genes involved in auxin metabolism (ILR, SAUR and ARF) and ABA metabolism (ZEP and PP2C) are upregulated in litchi aril, leading to differences in fruit development and pericarp mechanical strength (Table 5) (Wang et al., 2021a). Using the transcriptomic and metabolomics analysis, Wang et al. (2019a); Wang et al. (2019b) and Wang et al. (2021a) suggested that the susceptibility to litchi cracking may be associated with the difference in hormone balance of the two analyzed cultivars, that is, differences in metabolism of IAA (indoleacetic acid, natural auxin), ABA (abscisic acid), ethylene, BR (brassinosteroid) and JA (jasmonic acid) once the different metabolites generated by different hormone metabolism are cultivar specific and have distinct expression patterns in the three types of litchi pericarps. Thus, changes in gene expression and metabolites can explain the cracking susceptibility, namely a downregulation of OR1 and OR3 genes, and ACCS and H1 genes, involved in IAA and ethylene metabolisms, respectively, and an upregulation of CYP707A and TC/M genes involved in ABA and BR metabolisms, respectively, in cracking-resistant cultivar Feizixiao; in contrast, AOS (BR metabolism) gene present highest expression in cracked fruits from cracking-susceptible cultivar Baitangying (Table 5) (Wang et al., 2019a; Wang et al., 2019b). Also, in the ethylene metabolism associated to sucrose synthesis and sweetness increase, aA and SS genes are downregulated in a cracking-resistant cultivar while TLP gene is upregulated in cracked fruits from cracking-susceptible cultivar (Wang et al., 2019a; Wang et al., 2019b). Additionally, GH3 gene, involved in IAA, is upregulated in cracking-resistant cultivar (Wang et al., 2019a; Wang et al., 2019b; Wang et al., 2021a).
Cracking in atemoya seems to have a close relation with phytohormones, so, several hormones related genes has been identified. The majority is related to auxin and ABA pathways, with 18 and 12 genes, respectively, while cytokinin pathway presented 6 genes, salicylic acid pathway comprised 5 genes, gibberellin and jasmonic acid pathways included 3 genes and ethylene pathway only presented one gene. Comparing with fruits without cracks, the auxin and jasmonic acid related genes were downregulated in cracked fruits, while ABA, cytokinin, gibberellin, ethylene and salicylic acid related genes were upregulated, showing that these genes can have an important role in cracking (Li et al., 2019).
Transcription factors genes involved in fruit cracking
It’s known that different final products can be generated due to differential regulation by transcription factors according to environmental or development stimuli (Falginella et al., 2021).
In sweet cherry, WINA and WINB genes encode AP2/EREBP-type transcription factors, regulating several genes involved in cutin and wax biosynthesis (Table 6) (Alkio et al., 2012; Balbontín et al., 2014). According to Alkio et al. (2012), these genes present higher expression in the initial stage of fruit development. Balbontín et al. (2014) obtained similar results for WINB, finding higher expression levels in the beginning of fruit development in a cracking-resistant cultivar Kordia. This indicates that WINB can influence the expression of other cuticular waxes related genes during the fruit growth.
Likewise, a down-regulation of SHN3 leads to low cutin and wax contents and, consequently, to microcracks development in apple (Table 6) (Straube et al., 2021). SHN3 expression compromises cuticle formation, being considered an essential regulator of apple cuticle biosynthesis (Lashbrooke et al., 2015). Similar results were obtained by Falginella et al. (2021) in which high levels of SHN3, are related to high cutin and wax contents, and low russet development, while low levels of SHN3 transcripts leads to a decrease in cutin and wax contents as well as microcracks development and consequently, high russet development. In contrast, a lower expression of transcription factor MYB93, related to suberin and lignin synthesis, leads to low suberin content, resulting in low russet development, while a higher gene expression promotes an increase in suberin, allowing the microcracks development and, consequently, high russet development (Falginella et al., 2021) as also described by Straube et al. (2021) for the MYB93 and MYB42 genes (Table 6).
The ethylene-responsive transcription factor 4, ERF4, is considered the major gene underlying watermelon rind hardness regulation and thus an important factor in cracking resistance. However, beyond the function of ERF4 in rind hardness variability and consequently in cracking resistance, their expression can be affected by the regulation of other genes such as genes involved in cell wall modification and/or degradation as well as genes related to lignin biosynthesis (Liao et al., 2020). Moreover, MDP gene is upregulated in the cracking-resistant watermelon, while the expression level of HD-ZIP in cracking-resistant watermelon is lower than in cracking-susceptible watermelon (Jiang et al., 2019b).
In litchi, a decrease of the pericarp mechanical strength can occur due a downregulation of transcription factor genes, such as WRKY, bZIP, bHLH and MYB causing a development retardation in fruit and (Table 6) (Wang et al., 2021a). In contrast, an upregulation of transcription factor genes, like WRKY, bHLH, DOF and MYB cause an upregulation of starch/sucrose metabolism related genes and sugar/water transport and, thus, differences in mechanical strength of pericarp (Wang et al., 2021a).
Other potential genes/pathways involved in fruit cracking
During apple development, Joshi et al. (2018) found a significant upregulation in the majority of the genes during fruit development, suggesting that a high expression of cuticle-related genes can prevent crack initiation and possibly enhancing cuticular cracking repair.
Atemoya cracking can occur due to an unbalance among genes involved in hormone metabolisms (Li et al., 2019) as well as genes involved in starch metabolism and genes related to cell wall, whereby the transformation of starch into soluble sugars leads to an increase in turgor pressure and, consequently, in cells and tissues rupture. At the same time, the pectin and cellulose degradation decreases cell wall toughness, which together with starch metabolism leads to cracking development in the fruit pericarp (Chen et al., 2019a).
Furthermore, Zhang et al. (2021a) carried out an enrichment analysis during veraison and maturity stages using grapes that were treated with calcium as cracking mitigation strategy, to analyze the transcriptome and secondary metabolites in grape berry cracking. The enrichment analysis under the application of calcium sprays revealed that the main DEGs are related to flavone and flavonol biosynthesis pathway and also to flavonoid metabolism pathway, meaning that the balance of up and down regulation of genes involved in both pathways determine the grape berry cracking rate, and thus, allowing to identify new pathways and genes involved in grape berry cracking and also explain the role of calcium sprays in modulating these pathways and their effect in reducing cracking rate (Zhang et al., 2021a). However, Zhang et al. (2021a) suggest that other metabolic pathways can be involved in grape berry cracking.
In jujube, Hou et al. (2022) used cracked and non-cracked fruits of two cracking-susceptible cultivars, and non-cracked fruits of a cracking-resistant cultivar to elucidate cracking-related molecular mechanisms. Comparing samples from the cracking-resistant cultivar to samples with and without cracking of each cracking-susceptible cultivar, the authors found several cracking related genes which are involved in different metabolic pathways, namely in water transport, cell wall metabolism, starch and sucrose metabolism, cuticle structure, calcium transport, ABA metabolism, indoleacetic acid metabolism, jasmonic acid metabolism, gibberellic acid metabolism and transcription factors (Hou et al., 2022). In general, all cracking related genes involved in these pathways are upregulated in cracked fruits, compared to the non-cracked fruits and, thus, the authors propose that the high expression levels in cracked fruits leads to an increase in the turgor pressure and a decrease in the exocarp mechanical strength, which can lead to the fruit cracking development (Hou et al., 2022).
Importance for new molecular breeding
Genomics with the development of complete reference genomes, allows to find interesting molecular opportunities for the identification of candidate genes linked to agronomic traits (Bianchi et al., 2015; Soundararajan et al., 2019). Moreover, by the development of omics, such as metabolomics and transcriptomics, associated with whole-genome sequences provides great information about the molecular mechanisms in fruits (Zhang and Hao, 2020; Wang et al., 2021b). Thus, the combination of genomic, transcriptomic and metabolomic analyses can reveal important knowledge and genetic basis for crop’s molecular breeding (Zhang and Hao, 2020). Although the gene expression can be affected by internal and external factors, by the combined information provided by different omics, it is possible to identify transcription factors and key genes of different biosynthesis pathways as well as to understand how environmental conditions affects the traits of interest at molecular level, and consequently improve fruit quality and molecular breeding programs (García-Gómez et al., 2020).
Fruit trees are economically important, but the lengthy life cycles of several years slow the study at the genetic level. However, molecular tools represent a good strategy to understand adaptation to abiotic stresses and environmental conditions (Licciardello et al., 2021). So, it’s important to understand how thousands of genes can interact with each other as well as how the related metabolic pathways contribute to plant development and adaptation to the environment (Licciardello et al., 2021). Thus, the identification and characterization of genes controlling agricultural traits and tagging molecular markers constitutes advances for development of new breeding techniques (Soundararajan et al., 2019). In this follow up, the functional genomics in fruit trees has deployed several methodologies to improve the molecular breeding techniques in fruit crops, such as gene expression-based biomarkers, transcriptomic and metabolomics, whole-genome variations and sequence, among others (Licciardello et al., 2021). With the available information, several fruit quality traits can be improved, namely the development of new cultivars with small/larger size, good-flavored fruits, attractive color, sugar and acid levels, reduced juvenile phase, massive and constant yields, reduced susceptibility to fruit cracking, self-compatibility, and improved resistance or tolerance to disease as well as resistance to abiotic stresses like adverse environmental conditions (Soundararajan et al., 2019).
Regarding cracking, it’s known that there are several factors that affect fruit cracking, such as physiological, genetics, environmental and postharvest storage (Khadivi-Khub, 2015; Wang et al., 2021b). Among the several mitigation strategies to prevent cracking, calcium is a key mineral in plant physiology (Winkler and Knoche, 2021). It plays an important role in the pre- and postharvest physiology of most plant and particularly of fruit, being considered a critical nutrient in determining fruit quality (Winkler and Knoche, 2019). For example, calcium application in grape berry appears to induce specific modifications both in skin and in pulp, changing the cuticle structure, playing an important role in preventing cracking (Martins et al., 2020) as well as the inhibition of cell wall disassembly promoting cell wall strengthening and, thus, calcium can prevent grape cracking (Yu et al., 2020). Likewise, the use of calcium to mitigate the risk of pre-harvest rain-cracking of sweet cherry increases the fruit quality, firmness and shelf-life, reducing the cracking susceptibly (Correia et al., 2019; Winkler and Knoche, 2019; Breia et al., 2020; Winkler et al., 2020; Correia et al., 2020a; Matteo et al., 2022). Furthermore, in sweet cherry, the combination of calcium with growth regulators highly reduce the cracking incidence, promoting differential gene expression of some exocarp related genes (Correia et al., 2020b). Thus, a better knowledge about the cuticle related genes can provide new insights about molecular mechanisms involved in cracking of flesh fruits. For example, the understanding of exocarp development in sweet cherry as well as the expression of exocarp-specific genes during fruit growth, maturation, softening, cuticle deposition and sugar transport, can provide great information about their role in conferring cracking resistance (Alkio et al., 2014). Likewise, in watermelon, the identification of genes related to rind hardness and the associated molecular markers can help to understand rind hardness and fruit cracking resistance and, thus, used in future breeding programs, by CRISPR-Cas9 or marker-assisted selection, to create more resistant cultivars (Liao et al., 2020). Moreover, in jujube, the knowledge about the differences in the cuticular wax and the expression of related genes, using cultivars with different susceptibilities to cracking, may provide new insights about prevent cracking (Li et al., 2014), which coupled by possible enhancing or silencing of related genes by gene modification technology, can change the cell wall structure and arrangement, and, thus, help in fruit cracking prevention (Hou et al., 2018).
Actually, the effects of climate changes, like excessive rains, leads to different physiological responses of the crops, affecting fruit growth, development and quality (Hirpo and Gebeyehu, 2019). The fruit ripening, that is, from a green fruit to a ripe fruit, represents a synchronized process with changes in physiological structure and biochemical composition according to interactions among fruits and their environment (García-Gómez et al., 2020). Fruit cracking emerges as a physiological disorder during fruit development as response to genetic or environmental factors (Wang et al., 2021b). The fruit development is controlled by expression of several genes (polygenic expression regulated by hundreds to thousands of genes), with different associated molecular mechanisms. These include biochemical, transcriptional, hormonal or metabolites levels. Thus, when physiological disorders appear, like cracking, the different underlying mechanisms make it challenging to study (García-Gómez et al., 2020). However, in general, an increase of expression of genes related to cell wall mechanisms arises in cracking-resistant cultivars of sweet cherry (Balbontín et al., 2014; Michailidis et al., 2021), apple (Kasai et al., 2008; Joshi et al., 2018) or watermelon (Jiang et al., 2019b). This indicates a common mechanism causing cracking. Likewise, an upregulation of cuticular waxes related genes in apple (Gao et al., 2021; Straube et al., 2021), watermelon (Jiang et al., 2019b) or jujube (Li et al., 2021c) is also correlated with a decrease of cracking index. Moreover, an unbalance of genes related to hormone metabolisms can increase the cracking susceptibility in litchi (Wang et al., 2019a; Wang et al., 2019b; Wang et al., 2021a) and in atemoya (Li et al., 2019). Hou et al. (2022) reported several metabolic pathways involved in cracking jujube fruits, highlighting the complexity of this disorder.
Thus, the combination of genomic, transcriptomic and metabolomic analyses can reveal important knowledge and genetic basis for crops molecular breeding (Zhang and Hao, 2020). Moreover, the understanding about the differences in gene expression during fruit development elucidates the molecular mechanisms of cuticle related genes, playing important roles in preventing or enhancing fruit cracking (Hou et al., 2018).
Conclusion
Although fruit cracking remains a great challenge to the producers, it is known that the genetic factors play a crucial role in its development. The genetic component makes cracking an attractive field for researchers who work with molecular breeding. The development of different omics technologies, open news perspectives to understand how this disorder occurs at molecular level. The molecular mechanisms involved in cracking are based on correlations as direct proof of concept based on mutations or reverse genetics are still missing. It is known that exocarp-specific transcripts play a crucial role in cracking development, namely genes involved in cuticular membrane cuticular, cell wall mechanisms or cuticular wax biosynthesis. Additionally, by the analysis of the metabolome, which is closely related to phenotype, the regulation of several metabolic pathways can affect the expression of exocarp-specific genes and, consequently, affects the development of fruit cracking. This physiological disorder is enhanced by environmental conditions, such as high temperatures and heavy rain. The scenario of climate change, foreseen for the future, makes it even more urgent to understand the responses of plants to stress (simple or combined), at various levels, especially at the transcriptomic level. Many genes are repressed or induced in stress response, involving a precise regulation of complex stress-gene networks. It is therefore crucial to understand the function of the genes involved, to determine the functional relationships between genes and how they are affected by biotic or abiotic factors. Obtaining a set of candidate genes for molecular breeding programs by genome editing technologies should bring forward crop performance under changing environmental conditions.
Author contributions
MS, ME-C, BG and MM contributed to the conceptualization of the review. MS wrote the first draft of the manuscript. ME-C, BG and MM supervised, reviewed and edited the manuscript. All authors contributed to manuscript revision, read, and approved the submitted version.
Funding
This study was supported by the European Agricultural Fund for Rural Development (EAFRD) and by the Portuguese State in the context of action 1.1. Grupos Operacionais integrado na medida 1. Inovação do PDR 2020–Programa de Desenvolvimento Rural do Continente–Grupo Operacional para a valorização da produção da Cereja de Resende e posi-cionamento da subfileira nos mercados (iniciativa n° 362).
Acknowledgments
MS acknowledges the financial support provided by FCT - Portuguese Foundation for Science and Technology (PD/BD/150257/2019), under the Doctoral Program ‘Agricultural Production Chains – from fork to farm’ (PD/00122/2012). The authors also acknowledge the support of National Funds by FCT, under the project UIDB/04033/2020 (CITAB research unit).
Conflict of interest
The authors declare that the research was conducted in the absence of any commercial or financial relationships that could be construed as a potential conflict of interest.
Publisher’s note
All claims expressed in this article are solely those of the authors and do not necessarily represent those of their affiliated organizations, or those of the publisher, the editors and the reviewers. Any product that may be evaluated in this article, or claim that may be made by its manufacturer, is not guaranteed or endorsed by the publisher.
References
Alkio, M., Jonas, U., Declercq, M., Van Nocker, S., Knoche, M. (2014). Transcriptional dynamics of the developing sweet cherry (Prunus avium l.) fruit: Sequencing, annotation and expression profiling of exocarp-associated genes. Horticulture Res. 1 (1), 11. doi: 10.1038/hortres.2014.11
Alkio, M., Jonas, U., Sprink, T., van Nocker, S., Knoche, M. (2012). Identification of putative candidate genes involved in cuticle formation in Prunus avium (sweet cherry) fruit. Ann. Bot. 110 (1), 101–112. doi: 10.1093/aob/mcs087
Balbontín, C., Ayala, H., M. Bastías, R., Tapia, G., Ellena, M., Torres, C., et al. (2013). Cracking in sweet cherries: A comprehensive review from a physiological, molecular, and genomic perspective. Chilean J. Agric. Res. 73, 66–72. doi: 10.4067/S0718-58392013000100010
Balbontín, C., Ayala, H., Rubilar, J., Cote, J., Figueroa, C. R. (2014). Transcriptional analysis of cell wall and cuticle related genes during fruit development of two sweet cherry cultivars with contrasting levels of cracking tolerance. Chilean J. Agric. Res. 74, 162–169. doi: 10.4067/S0718-58392014000200006
Bargel, H., Neinhuis, C. (2005). Tomato (Lycopersicon esculentum mill.) fruit growth and ripening as related to the biomechanical properties of fruit skin and isolated cuticle. J. Exp. Bot. 56 (413), 1049–1060. doi: 10.1093/jxb/eri098
Beyer, M., Lau, S., Knoche, M. (2005). Studies on water transport through the sweet cherry fruit surface: IX. comparing permeability in water uptake and transpiration. Planta 220 (3), 474–485. doi: 10.1007/s00425-004-1354-y
Bianchi, V. J., Rubio, M., Trainotti, L., Verde, I., Bonghi, C., Martínez-Gómez, P. (2015). Prunus transcription factors: breeding perspectives. Front. Plant Sci. 6 (443). doi: 10.3389/fpls.2015.00443
Breia, R., Mósca, A. F., Conde, A., Correia, S., Conde, C., Noronha, H., et al. (2020). Sweet cherry (Prunus avium l.) PaPIP1;4 is a functional aquaporin upregulated by pre-harvest calcium treatments that prevent cracking. Int. J. Mol. Sci. 21 (8), 3017. doi: 10.3390/ijms21083017
Brüggenwirth, M., Knoche, M. (2017). Cell wall swelling, fracture mode, and the mechanical properties of cherry fruit skins are closely related. Planta 245 (4), 765–777. doi: 10.1007/s00425-016-2639-7
Brummell, D. A. (2006). Primary cell wall metabolism during fruit ripening. New Z. J. Forestry Sci. 36 (1), 99–111.
Brummell, D. A., Harpster, M. H. (2001). Cell wall metabolism in fruit softening and quality and its manipulation in transgenic plants. Plant Mol. Biol. 47 (1), 311–339. doi: 10.1023/A:1010656104304
Brummell, D. A., Harpster, M. H., Civello, P. M., Palys, J. M., Bennett, A. B., Dunsmuir, P. (1999). Modification of expansin protein abundance in tomato fruit alters softening and cell wall polymer metabolism during ripening. Plant Cell 11 (11), 2203–2216. doi: 10.1105/tpc.11.11.2203
Butani, A., Purohit, H., Solanki, R., Mishra, P., Dadhaniya, D. (2019). A chronic problem of fruit cracking in fruit crops: A review. Acta Sci. Agric. 3 (4), 270–274.
Chen, J., Duan, Y., Hu, Y., Li, W., Sun, D., Hu, H., et al. (2019a). Transcriptome analysis of atemoya pericarp elucidates the role of polysaccharide metabolism in fruit ripening and cracking after harvest. BMC Plant Biol. 19 (1), 219. doi: 10.1186/s12870-019-1756-4
Chen, Y.-H., Khanal, B. P., Linde, M., Debener, T., Alkio, M., Knoche, M. (2019b). Expression of putative aquaporin genes in sweet cherry is higher in flesh than skin and most are downregulated during development. Scientia Hortic. 244, 304–314. doi: 10.1016/j.scienta.2018.09.065
Christensen, J. V. (1972). Cracking in cherries. Acta Agriculturae Scandinavica 22 (2), 128–136. doi: 10.1080/00015127209433471
Correia, S., Queirós, F., Ferreira, H., Morais, M. C., Afonso, S., Silva, A. P., et al. (2020a). Foliar application of calcium and growth regulators modulate sweet cherry (Prunus avium l.) tree performance. Plants (Basel) 9 (4), 410. doi: 10.3390/plants9040410
Correia, S., Queirós, F., Ribeiro, C., Vilela, A., Aires, A., Barros, A. I., et al. (2019). Effects of calcium and growth regulators on sweet cherry (Prunus avium l.) quality and sensory attributes at harvest. Scientia Hortic. 248, 231–240. doi: 10.1016/j.scienta.2019.01.024
Correia, S., Santos, M., Glińska, S., Gapińska, M., Matos, M., Carnide, V., et al. (2020b). Effects of exogenous compound sprays on cherry cracking: Skin properties and gene expression. J. Sci. Food Agric. 100 (7), 2911–2921. doi: 10.1002/jsfa.10318
Correia, S., Schouten, R., Silva, A. P., Gonçalves, B. (2018). Sweet cherry fruit cracking mechanisms and prevention strategies: A review. Scientia Hortic. 240, 369–377. doi: 10.1016/j.scienta.2018.06.042
Declercq, M., Alkio, M., Sprink, T., Schreiber, L., Knoche, M. J. T. G., Genomes (2014). Effect of sweet cherry genes PaLACS2 and PaATT1 on cuticle deposition, composition and permeability in Arabidopsis. Tree Genet. Genomes 10, 1711–1721. doi: 10.1007/s11295-014-0791-4
Domínguez, E., Fernández, M. D., Hernández, J. C. L., Parra, J. P., España, L., Heredia, A., et al. (2012). Tomato fruit continues growing while ripening, affecting cuticle properties and cracking. Physiol. Plantarum 146 (4), 473–486. doi: 10.1111/j.1399-3054.2012.01647.x
Falginella, L., Andre, C. M., Legay, S., Lin-Wang, K., Dare, A. P., Deng, C., et al. (2021). Differential regulation of triterpene biosynthesis induced by an early failure in cuticle formation in apple. Horticulture Res. 8 (1), 75. doi: 10.1038/s41438-021-00511-4
Franck, C., Lammertyn, J., Ho, Q. T., Verboven, P., Verlinden, B., Nicolaï, B. M. (2007). Browning disorders in pear fruit. Postharvest Biol. Technol. 43 (1), 1–13. doi: 10.1016/j.postharvbio.2006.08.008
Franco-Zorrilla, J. M., López-Vidriero, I., Carrasco, J. L., Godoy, M., Vera, P., Solano, R. (2014). DNA-binding specificities of plant transcription factors and their potential to define target genes. Proc. oh Natl. Acad. Sci. 111 (6), 2367–2372. doi: 10.1073/pnas.1316278111
Gao, H. N., Jiang, H., Lian, X. Y., Cui, J. Y., You, C. X., Hao, Y. J., et al. (2021). Identification and functional analysis of the MdLTPG gene family in apple. Plant Physiol. Biochem. 163, 338–347. doi: 10.1016/j.plaphy.2021.04.015
García-Gómez, B. E., Salazar, J. A., Nicolás-Almansa, M., Razi, M., Rubio, M., Ruiz, D., et al. (2020). Molecular bases of fruit quality in Prunus species: An integrated genomic, transcriptomic, and metabolic review with a breeding perspective. Int. J. Mol. Sci. 22 (1), 333. doi: 10.3390/ijms22010333
Gonzali, S., Perata, P. (2021). Fruit colour and novel mechanisms of genetic regulation of pigment production in tomato fruits. Horticulturae 7 (8), 259. doi: 10.3390/horticulturae7080259
Goulao, L. F., Oliveira, C. M. (2008). Cell wall modifications during fruit ripening: when a fruit is not the fruit. Trends Food Sci. Technol. 19 (1), 4–25. doi: 10.1016/j.tifs.2007.07.002
Hirpo, F. H., Gebeyehu, M. N. (2019). Review on the effects of climate change variability on horticultural productivity. Int. J. Environ. Sci. Natural Resour. 17 (4), 555969. doi: 10.19080/IJESNR.2019.17.555969
Hou, L., Li, M., Zhang, C., Liu, N., Liu, X., Bo, W., et al. (2022). Comparative transcriptomic analyses of different jujube cultivars reveal the Co-regulation of multiple pathways during fruit cracking. Genes 13 (1), 105. doi: 10.3390/genes13010105
Hou, S. Y., Shen, J., Sun, Z. X., Li, H. Y. (2018). Identification of genes related to cell wall metabolism and fruit ripening in Ziziphus jujube using RNA-seq and expression analysis. Russian J. Plant Physiol. 65 (4), 604–610. doi: 10.1134/S102144371804012X
Hovav, R., Chehanovsky, N., Moy, M., Jetter, R., Schaffer, A. A. (2007). The identification of a gene (Cwp1), silenced during Solanum evolution, which causes cuticle microfissuring and dehydration when expressed in tomato fruit. Plant J. 52 (4), 627–639. doi: 10.1111/j.1365-313X.2007.03265.x
Javed, T., Shabbir, R., Ali, A., Afzal, I., Zaheer, U., Gao, S. J. (2020). Transcription factors in plant stress responses: Challenges and potential for sugarcane improvement. Plants 9, 491. doi: 10.3390/plants9040491
Jiang, Y., Duan, X., Joyce, D., Zhang, Z., Li, J. (2004). Advances in understanding of enzymatic browning in harvested litchi fruit. Food Chem. 88 (3), 443–446. doi: 10.1016/j.foodchem.2004.02.004
Jiang, F., Lopez, A., Jeon, S., de Freitas, S. T., Yu, Q., Wu, Z., et al. (2019a). Disassembly of the fruit cell wall by the ripening-associated polygalacturonase and expansin influences tomato cracking. Horticulture Res. 6 (1), 17. doi: 10.1038/s41438-018-0105-3
Jiang, H., Tian, H., Yan, C., Jia, L., Wang, Y., Wang, M., et al. (2019b). RNA-seq analysis of watermelon (Citrullus lanatus) to identify genes involved in fruit cracking. Scientia Hortic. 248, 248–255. doi: 10.1016/j.scienta.2019.01.005
Joshi, M., Baghel, R. S., Fogelman, E., Stern, R. A., Ginzberg, I. (2018). Identification of candidate genes mediating apple fruit-cracking resistance following the application of gibberellic acids 4+ 7 and the cytokinin 6-benzyladenine. Plant Physiol. Biochem. 127, 436–445. doi: 10.1016/j.plaphy.2018.04.015
Joshi, R., Wani, S. H., Singh, B., Bohra, A., Dar, Z. A., Lone, A. A., et al. (2016). Transcription factors and plants response to drought stress: Current understanding and future directions. Front. Plant Sci. 7. doi: 10.3389/fpls.2016.01029
Kasai, S., Hayama, H., Kashimura, Y., Kudo, S., Osanai, Y. (2008). Relationship between fruit cracking and expression of the expansin gene MdEXPA3 in ‘Fuji’apples (Malus domestica borkh.). Scientia Hortic. 116 (2), 194–198. doi: 10.1016/j.scienta.2007.12.002
Kaur, G. (2019). Review of XET enzymes, current applications and future trends. Int. J. Latest Technol. Engineering Manage. Appl. Sci. 8, 52–55.
Khadivi-Khub, A. (2015). Physiological and genetic factors influencing fruit cracking. Acta Physiologiae Plantarum 37 (1), 1718. doi: 10.1007/s11738-014-1718-2
Knoche, M., Khanal, B. P., Stopar, M. (2011). Russeting and microcracking of ‘Golden delicious’ apple fruit concomitantly decline due to gibberellin A4+7 application. J. Am. Soc. Hortic. Sci. 136 (3), 159–164. doi: 10.21273/jashs.136.3.159
Knoche, M., Lang, A. (2017). Ongoing growth challenges fruit skin integrity. Crit. Rev. Plant Sci. 36 (3), 190–215. doi: 10.1080/07352689.2017.1369333
Knoche, M., Peschel, S. (2002). Studies on water transport through the sweet cherry fruit surface. VI. effect of hydrostatic pressure on water uptake. J. Hortic. Sci. Biotechnol. 77 (5), 609–614. doi: 10.1080/14620316.2002.11511546
Knoche, M., Winkler, A. (2019). The mechanism of rain cracking of sweet cherry fruit. Italus Hortus 26 (1), 59–65. doi: 10.26353/j.itahort/2019.1.5965
Kovács, E., Kristóf, Z., Perlaki, R., Szőllősi, D. (2008). Cell wall metabolism during ripening and storage of nonclimacteric sour cherry (Prunus cerasus l., cv. kántorjánosi). Acta Alimentaria 37 (4), 415–426. doi: 10.1556/aalim.2008.0011
Lane, W. D., Meheriuk, M., McKenzie, D.-L. (2000). Fruit cracking of a susceptible, an intermediate, and a resistant sweet cherry cultivar. HortScience 35 (2), 239–242. doi: 10.21273/hortsci.35.2.239
Lara, I., Belge, B., Goulao, L. F. (2014). The fruit cuticle as a modulator of postharvest quality. Postharvest Biol. Technol. 87, 103–112. doi: 10.1016/j.postharvbio.2013.08.012
Lara, I., Heredia, A., Domínguez, E. (2019). Shelf life potential and the fruit cuticle: The unexpected player. Front. Plant Sci. 10. doi: 10.3389/fpls.2019.00770
Lashbrooke, J., Aharoni, A., Costa, F. (2015). Genome investigation suggests MdSHN3, an APETALA2-domain transcription factor gene, to be a positive regulator of apple fruit cuticle formation and an inhibitor of russet development. J. Exp. Bot. 66 (21), 6579–6589. doi: 10.1093/jxb/erv366
Le Gall, H., Philippe, F., Domon, J. M., Gillet, F., Pelloux, J., Rayon, C. (2015). Cell wall metabolism in response to abiotic stress. Plants 4 (1), 112–166. doi: 10.3390/plants4010112
Li, N., Fu, L., Song, Y., Li, J., Xue, X., Li, S., et al. (2020). Wax composition and concentration in jujube (Ziziphus jujuba mill.) cultivars with differential resistance to fruit cracking. J. Plant Physiol. 255, 153294. doi: 10.1016/j.jplph.2020.153294
Li, Y., Jones, L., McQueen-Mason, S. (2003). Expansins and cell growth. Curr. Opin. Plant Biol. 6 (6), 603–610. doi: 10.1016/j.pbi.2003.09.003
Li, N., Li, X. Z., Song, Y. Q., Yang, S. T., Li, L. L. (2021b). Genome-wide identification, characterization, and expression profiling of the ECERIFERUM (CER) gene family in Ziziphus jujube. Russian J. Plant Physiol. 68 (5), 828–837. doi: 10.1134/S1021443721050101
Li, H., Li, W., Zhang, T., Zhong, J., Liu, J., Yuan, C., et al. (2019). Comparative transcriptomic analysis of split and non-split atemoya (Annona cherimola mill. × Annona squamosa l.) fruit to identify potential genes involved in the fruit splitting process. Scientia Hortic. 248, 216–224. doi: 10.1016/j.scienta.2019.01.017
Li, H., Liu, G., Tian, H., Fu, D. (2021a). [Fruit cracking: a review]. Sheng Wu Gong Cheng Xue Bao 37 (8), 2737–2752. doi: 10.13345/j.cjb.200553
Li, N., Song, Y., Li, J., Hao, R., Feng, X., Li, L. (2021c). Transcriptome and genome re-sequencing analysis reveals differential expression patterns and sequence variation in pericarp wax metabolism-related genes in Ziziphus jujuba (Chinese jujube). Scientia Hortic. 288, 110415. doi: 10.1016/j.scienta.2021.110415
Li, W.-C., Wu, J.-Y., Zhang, H.-N., Shi, S.-Y., Liu, L.-Q., Shu, B., et al. (2014). De novo assembly and characterization of pericarp transcriptome and identification of candidate genes mediating fruit cracking in Litchi chinensis sonn. Int. J. Mol. Sci. 15 (10), 17667–17685. doi: 10.3390/ijms151017667
Liao, N., Hu, Z., Li, Y., Hao, J., Chen, S., Xue, Q., et al. (2020). Ethylene-responsive factor 4 is associated with the desirable rind hardness trait conferring cracking resistance in fresh fruits of watermelon. Plant Biotechnol. J. 18 (4), 1066–1077. doi: 10.1111/pbi.13276
Licciardello, C., Perrone, I., Gambino, G., Talon, M., Velasco, R. (2021). Editorial: Functional genomics in fruit trees: From ‘Omics to sustainable biotechnologies. Front. Plant Sci. 12(1518), doi: 10.3389/fpls.2021.729714
Liu, Y., Zhang, P., Geng, Y., Xie, X., Wen, P. (2020). Cracking of jujube fruits is associated with differential expression of metabolic genes. FEBS Open Bio 10 (9), 1765–1773. doi: 10.1002/2211-5463.12925
Lu, W., Wang, Y., Jiang, Y., Li, J., Liu, H., Duan, X., et al. (2006). Differential expression of litchi XET genes in relation to fruit growth. Plant Physiol. Biochem. 44 (11-12), 707–713. doi: 10.1016/j.plaphy.2006.09.020
Macnee, N. C., Rebstock, R., Hallett, I. C., Schaffer, R. J., Bulley, S. M. (2020). A review of current knowledge about the formation of native peridermal exocarp in fruit. Funct. Plant Biol. 47 (12), 1019–1031. doi: 10.1071/fp19135
Marboh, E. S., Singh, S. K., Swapnil, P., Nath, V., Gupta, A. K., Pongener, A. (2017). Fruit cracking in litchi (Litchi chinensis): An overview. Indian J. Agric. Sci. 87 (1), 3–11.
Marowa, P., Ding, A., Kong, Y. (2016). Expansins: roles in plant growth and potential applications in crop improvement. Plant Cell Rep. 35 (5), 949–965. doi: 10.1007/s00299-016-1948-4
Martins, V., Garcia, A., Alhinho, A. T., Costa, P., Lanceros-Méndez, S., Costa, M. M. R., et al. (2020). Vineyard calcium sprays induce changes in grape berry skin, firmness, cell wall composition and expression of cell wall-related genes. Plant Physiol. Biochem. 150, 49–55. doi: 10.1016/j.plaphy.2020.02.033
Matteo, M., Zoffoli, J. P., Ayala, M. (2022). Calcium sprays and crop load reduction increase fruit quality and postharvest storage in sweet cherry (Prunus avium l.). Agronomy 12 (4), 829. doi: 10.3390/agronomy12040829
Michailidis, M., Karagiannis, E., Bazakos, C., Tanou, G., Ganopoulos, I., Molassiotis, A. (2021). Genotype- and tissue-specific metabolic networks and hub genes involved in water-induced distinct sweet cherry fruit cracking phenotypes. Comput. Struct. Biotechnol. J. 19, 5406–5420. doi: 10.1016/j.csbj.2021.09.030
Mintz-Oron, S., Mandel, T., Rogachev, I., Feldberg, L., Lotan, O., Yativ, M., et al. (2008). Gene expression and metabolism in tomato fruit surface tissues. Plant Physiol. 147 (2), 823–851. doi: 10.1104/pp.108.116004
Moctezuma, E., Smith, D. L., Gross, K. C. (2003). Antisense suppression of a β-galactosidase gene (TBG6) in tomato increases fruit cracking. J. Exp. Bot. 54 (390), 2025–2033. doi: 10.1093/jxb/erg214
Peschel, S., Knoche, M. (2012). Studies on water transport through the sweet cherry fruit surface: XII. variation in cuticle properties among cultivars. J. Am. Soc. Hortic. Sci. 137 (6), 367–375. doi: 10.21273/JASHS.137.6.367
Petit, J., Bres, C., Just, D., Garcia, V., Mauxion, J. P., Marion, D., et al. (2014). Analyses of tomato fruit brightness mutants uncover both cutin-deficient and cutin-abundant mutants and a new hypomorphic allele of GDSL lipase. Plant Physiol. 164 (2), 888–906. doi: 10.1104/pp.113.232645
Petit, J., Bres, C., Mauxion, J. P., Bakan, B., Rothan, C. (2017). Breeding for cuticle-associated traits in crop species: traits, targets, and strategies. J. Exp. Bot. 68 (19), 5369–5387. doi: 10.1093/jxb/erx341
Rehman, M. U., Rather, G. H., Dar, N. A., Mir, M. M., Iqbal, U., Mir, M. R., et al. (2015). Causes and prevention of cherry cracking: A review, in Crop production and global environmental issues. Ed. Hakeem., K. R. (Cham: Springer International Publishing), 543–552. doi: 10.1007/978-3-319-23162-4_19
Ren, Y.-x., Shen, L., Wang, X.-l., Yan, C., Mao, L., Mao, Y. M. (2017). Study of the related cracking-resistant genes in chinese jujube, Scientific Papers. Series B, Horticulture vol. LXI:155–164. (S. P. S. B. Horticulture).
Romero, P., Rose, J. K. C. (2019). A relationship between tomato fruit softening, cuticle properties and water availability. Food Chem. 295, 300–310. doi: 10.1016/j.foodchem.2019.05.118
Sahadev, D. R., Vikas, U., Sachin, D. P., Sharad, R. B. (2017). Berry cracking; its causes and remedies in grapes - a review. Trends Biosci. 10 (2), 549–556.
Samuels, L., Kunst, L., Jetter, R. (2008). Sealing plant surfaces: cuticular wax formation by epidermal cells. Annu. Rev. Plant Biol. 59, 683–707. doi: 10.1146/annurev.arplant.59.103006.093219
Schumann, C., Winkler, A., Brüggenwirth, M., Köpcke, K., Knoche, M. (2019). Crack initiation and propagation in sweet cherry skin: A simple chain reaction causes the crack to 'run'. PloS One 14 (7), e0219794. doi: 10.1371/journal.pone.0219794
Shahzad, R., Jamil, S., Ahmad, S., Nisar, A., Amina, Z., Saleem, S., et al. (2021). Harnessing the potential of plant transcription factors in developing climate resilient crops to improve global food security: Current and future perspectives. Saudi J. Biol. Sci. 28 (4), 2323–2341. doi: 10.1016/j.sjbs.2021.01.028
Simon, G. (2006). Review on rain induced fruit cracking of sweet cherries (Prunus avium l.), its causes and the possibilities of prevention. Int. J. Hortic. Sci. 12 (3), 27–35. doi: 10.31421/IJHS/12/3/654
Simon, G., Hrotkó, K., Magyar, L. (2004). Fruit quality of sweet cherry cultivars grafted on four different rootstocks. Acta Hortic. 658, 365–370. doi: 10.17660/ActaHortic.2004.658.53
Singh, A., Shukla, A. K., Meghwal, P. R. (2020). Fruit cracking in pomegranate: Extent, cause, and management – a review. Int. J. Fruit Sci. 20 (sup3), S1234–S1253. doi: 10.1080/15538362.2020.1784074
Smith, D. L., Abbott, J. A., Gross, K. C. (2002). Down-regulation of tomato β-galactosidase 4 results in decreased fruit softening. Plant Physiol. 129 (4), 1755–1762. doi: 10.1104/pp.011025
Soundararajan, P., Won, S. Y., Kim, J. S. (2019). Insight on rosaceae family with genome sequencing and functional genomics perspective. BioMed. Res. Int. 2019 (3), 7519687. doi: 10.1155/2019/7519687
Stratilová, B., Kozmon, S., Stratilová, E., Hrmova, M. (2020). Plant xyloglucan xyloglucosyl transferases and the cell wall structure: Subtle but significant. Molecules 25 (23), 5619. doi: 10.3390/molecules25235619
Straube, J., Chen, Y.-H., Khanal, B. P., Shumbusho, A., Zeisler-Diehl, V., Suresh, K., et al. (2021). Russeting in apple is initiated after exposure to moisture ends: Molecular and biochemical evidence. Plants 10 (1), 65. doi: 10.3390/plants10010065
Teh, H. F., Neoh, B. K., Wong, Y. C., Kwong, Q. B., Ooi, T. E. K., Ng, T. L. M., et al. (2014). Hormones, polyamines, and cell wall metabolism during oil palm fruit mesocarp development and ripening. J. Agric. Food Chem. 62 (32), 8143–8152. doi: 10.1021/jf500975h
Thimmappa, R., Geisler, K., Louveau, T., O'Maille, P., Osbourn, A. (2014). Triterpene biosynthesis in plants. Annu. Rev. Plant Biol. 65 (1), 225–257. doi: 10.1146/annurev-arplant-050312-120229
Trivedi, P., Nguyen, N., Hykkerud, A. L., Häggman, H., Martinussen, I., Jaakola, L., et al. (2019). Developmental and environmental regulation of cuticular wax biosynthesis in fleshy fruits. Front. Plant Sci. 10. doi: 10.3389/fpls.2019.00431
Vogg, G., Fischer, S., Leide, J., Emmanuel, E., Jetter, R., Levy, A. A., et al. (2004). Tomato fruit cuticular waxes and their effects on transpiration barrier properties: functional characterization of a mutant deficient in a very-long-chain fatty acid β-ketoacyl-CoA synthase. J. Exp. Bot. 55 (401), 1401–1410. doi: 10.1093/jxb/erh149
Wakasa, Y., Hatsuyama, Y., Takahashi, A., Sato, T., Niizeki, M., Harada, T. (2003). Divergent expression of six expansin genes during apple fruit ontogeny. Eur. J. Hortic. Sci. 68 (6), 253–259.
Wang, J., Gao, X., Ma, Z., Chen, J., Liu, Y. (2019a). Analysis of the molecular basis of fruit cracking susceptibility in Litchi chinensis cv. baitangying by transcriptome and quantitative proteome profiling. J. Plant Physiol. 234-235, 106–116. doi: 10.1016/j.jplph.2019.01.014
Wang, J. G., Gao, X. M., Ma, Z. L., Chen, J., Liu, Y. N., Shi, W. Q. (2019b). Metabolomic and transcriptomic profiling of three types of litchi pericarps reveals that changes in the hormone balance constitute the molecular basis of the fruit cracking susceptibility of Litchi chinensis cv. baitangying. Mol. Biol. Rep. 46 (5), 5295–5308. doi: 10.1007/s11033-019-04986-2
Wang, Y., Guo, L., Zhao, X., Zhao, Y., Hao, Z., Luo, H., et al. (2021b). Advances in mechanisms and omics pertaining to fruit cracking in horticultural plants. Agronomy 11 (6), 1045. doi: 10.3390/agronomy11061045
Wang, J., Wu, X. F., Tang, Y., Li, J. G., Zhao, M. L. (2021a). RNA-seq provides new insights into the molecular events involved in "Ball-skin versus bladder effect" on fruit cracking in litchi. Int. J. Mol. Sci. 22 (1), 454. doi: 10.3390/ijms22010454
Weichert, H., Knoche, M. (2006). Studies on water transport through the sweet cherry fruit surface. 10. evidence for polar pathways across the exocarp. J. Agric. Food Chem. 54 (11), 3951–3958. doi: 10.1021/jf053220a
Winkler, A., Fiedler, B., Knoche, M. (2020). Calcium physiology of sweet cherry fruits. Trees 34 (5), 1157–1167. doi: 10.1007/s00468-020-01986-9
Winkler, A., Knoche, M. (2019). Calcium and the physiology of sweet cherries: A review. Scientia Hortic. 245, 107–115. doi: 10.1016/j.scienta.2018.10.012
Winkler, A., Knoche, M. (2021). Calcium uptake through skins of sweet cherry fruit: Effects of different calcium salts and surfactants. Scientia Hortic. 276, 109761. doi: 10.1016/j.scienta.2020.109761
Winkler, A., Peschel, S., Kohrs, K., Knoche, M. (2016). Rain cracking in sweet cherries is not due to excess water uptake but to localized skin phenomena. J. Am. Soc. Hortic. Sci. 141 (6), 653–660. doi: 10.21273/JASHS03937-16
Xue, L., Sun, M., Wu, Z., Yu, L., Yu, Q., Tang, Y., et al. (2020). LncRNA regulates tomato fruit cracking by coordinating gene expression via a hormone-redox-cell wall network. BMC Plant Biol. 20 (1), 162. doi: 10.1186/s12870-020-02373-9
Yang, H., Liu, J., Dang, M., Zhang, B., Li, H., Meng, R., et al. (2018). Analysis of β-galactosidase during fruit development and ripening in two different texture types of apple cultivars. Front. Plant Sci. 9. doi: 10.3389/fpls.2018.00539
Yong, W., Wangjin, L., Jianguo, L., Yueming, J. (2006). Differential expression of two expansin genes in developing fruit of cracking-susceptible and-resistant litchi cultivars. J. Am. Soc. Hortic. Sci. 131 (1), 118–121. doi: 10.21273/JASHS.131.1.118
Yu, J., Zhu, M., Bai, M., Xu, Y., Fan, S., Yang, G. (2020). Effect of calcium on relieving berry cracking in grape (Vitis vinifera l.) 'Xiangfei'. PeerJ 8, e9896. doi: 10.7717/peerj.9896
Zarrouk, O., Pinheiro, C., Misra, C. S., Fernández, V., Chaves, M. M. (2018). “Chapter 20 - fleshy fruit epidermis is a protective barrier under water stress,” in Water scarcity and sustainable agriculture in semiarid environment. Eds. Tejero, I.F.García, Zuazo, V.H.Durán (Academic Press), Cambridge, MA, USA 507–533. doi: 10.1016/B978-0-12-813164-0.00020-X
Zhang, C., Cui, L., Zhang, P., Dong, T., Fang, J. (2021a). Transcriptome and metabolite profiling reveal that spraying calcium fertilizer reduces grape berry cracking by modulating the flavonoid biosynthetic metabolic pathway. Food Chemistry: Mol. Sci. 2, 100025. doi: 10.1016/j.fochms.2021.100025
Zhang, C., Hao, Y.-J. (2020). Advances in genomic, transcriptomic, and metabolomic analyses of fruit quality in fruit crops. Hortic. Plant J. 6 (6), 361–371. doi: 10.1016/j.hpj.2020.11.001
Zhang, C., Wang, T., Li, J., Zhang, D., Xie, Q., Munir, S., et al. (2021b). Functional gain of fruit netted-cracking in an introgression line of tomato with higher expression of the FNC gene. Front. Agric. Sci. Eng. 8 (2), 280–291. doi: 10.15302/j-fase-2020374
Zhang, P., Zhang, H., Du, J., Qiao, Y. (2022). Genome-wide identification and co-expression analysis of GDSL genes related to suberin formation during fruit russeting in pear. Hortic. Plant J. 8 (2), 153–170. doi: 10.1016/j.hpj.2021.11.010
Zhang, J., Zhang, Y.-F., Zhang, P.-F., Bian, Y.-H., Liu, Z.-Y., Zhang, C., et al. (2020). An integrated metabolic and transcriptomic analysis reveals the mechanism through which fruit bagging alleviates exocarp semi-russeting in pear fruit. Tree Physiol. 41 (7), 1306–1318. doi: 10.1093/treephys/tpaa172
Keywords: environmental stress, exocarp-specific genes, fruit cracking, gene expression, molecular mechanisms
Citation: Santos M, Egea-Cortines M, Gonçalves B and Matos M (2023) Molecular mechanisms involved in fruit cracking: A review. Front. Plant Sci. 14:1130857. doi: 10.3389/fpls.2023.1130857
Received: 23 December 2022; Accepted: 08 February 2023;
Published: 01 March 2023.
Edited by:
Sara Álvarez, Instituto Tecnológico Agrario de Castilla y León, SpainReviewed by:
Michail Michailidis, Aristotle University of Thessaloniki, GreeceMintao Sun, CAAS, China
Evangelos Karagiannis, University of Western Macedonia, Greece
Copyright © 2023 Santos, Egea-Cortines, Gonçalves and Matos. This is an open-access article distributed under the terms of the Creative Commons Attribution License (CC BY). The use, distribution or reproduction in other forums is permitted, provided the original author(s) and the copyright owner(s) are credited and that the original publication in this journal is cited, in accordance with accepted academic practice. No use, distribution or reproduction is permitted which does not comply with these terms.
*Correspondence: Marlene Santos, bXBzYW50b3NAdXRhZC5wdA==