- 1Department of Breast Surgery, Breast Disease Center, Affiliated Qingdao Central Hospital, Qingdao University, Qingdao, China
- 2Department of Oncology, Affiliated Qingdao Central Hospital, Qingdao University, Qingdao, China
- 3Department of Acupuncture and Moxibustion, Affiliated Qingdao Central Hospital, Qingdao University, Qingdao, China
- 4Department of Radiology, Affiliated Qingdao Central Hospital, Qingdao University, Qingdao, China
- 5Department of Gynecological Neoplasms, Affiliated Qingdao Central Hospital, Qingdao University, Qingdao, China
Flavonoids are one of the most important bioactive components in litchi (Litchi chinensis Sonn.) seeds and have broad-spectrum antiviral and antitumor activities. Litchi seeds have been shown to inhibit the proliferation of cancer cells and induce apoptosis, particularly effective against breast and liver cancers. Elucidating the distribution of flavonoids is important for understanding their physiological and biochemical functions and facilitating their efficient extraction and utilization. However, the spatial distribution patterns and expression states of flavonoids in litchi seeds remain unclear. Herein, matrix-assisted laser desorption/ionization mass spectrometry imaging (MALDI-MSI) was used for in situ detection and imaging of the distribution of flavonoids in litchi seed tissue sections for the first time. Fifteen flavonoid ion signals, including liquiritigenin, apigenin, naringenin, luteolin, dihydrokaempferol, daidzein, quercetin, taxifolin, kaempferol, isorhamnetin, myricetin, catechin, quercetin 3-β-d-glucoside, baicalin, and rutin, were successfully detected and imaged in situ through MALDI-MSI in the positive ion mode using 2-mercaptobenzothiazole as a matrix. The results clearly showed the heterogeneous distribution of flavonoids, indicating the potential of litchi seeds for flavonoid compound extraction. MALDI-MS-based multi-imaging enhanced the visualization of spatial distribution and expression states of flavonoids. Thus, apart from improving our understanding of the spatial distribution of flavonoids in litchi seeds, our findings also facilitate the development of MALDI-MSI-based metabolomics as a novel effective molecular imaging tool for evaluating the spatial distribution of endogenous compounds.
Introduction
Litchi (Litchi chinensis Sonn.; order Sapindales, family Sapindaceae), also known as Lizhi, Danli, and Liguo, is a subtropical fruit tree with a cultivation history in China of more than 2,300 years (Hu et al., 2021). It is the only species of Litchi (Yao et al., 2021). Litchi is an important fruit crop in southern China and is planted on more than 550,000 ha with an annual output of more than 2.2 million tons (Hu et al., 2021). The cultivation area and output of litchi in China account for more than 60% of global production (Li et al., 2020). Litchi seeds are a major product, but only a small portion is processed for biological utilization, and many litchi seeds are discarded as waste. The abandonment of fruit seed residues is not only a considerable problem for the environment but also a waste of global resources. Litchi seeds are rich in various bioactive compounds, such as flavonoids, saponins, volatile oils, polyols, alkaloids, steroids, coumarins, fatty acids, amino acids, and sugars (Dong et al., 2019; Punia and Kumar, 2021), resulting in a variety of biological functions, including antiviral and anti-oxidation activities, reducing the degree of liver damage and lowering blood glucose levels (Choi et al., 2017; Dong et al., 2019; Punia and Kumar, 2021). Accumulating evidence has confirmed the antitumor/anticancer effects of litchi seed extracts (Emanuele et al., 2017; Tang et al., 2018; Zhao et al., 2020).
Flavonoids are polyphenolic compounds and endogenous bioactive components, which act as secondary metabolites with extensive pharmacological activities. Flavonoids exert important pharmacological properties, including cardioprotective, anticancer, anti-inflammatory, and anti-allergic activities (Maleki et al., 2019; Ciumărnean et al., 2020; Liskova et al., 2021; Rakha et al., 2022). Regarding anticancer activity, many preclinical studies indicated the antiproliferative effects of flavonoids on lung (Berk et al., 2022), prostate (Vue et al., 2016), colorectal (Park et al., 2012; Li et al., 2018b), and breast (Pan et al., 2012) cancers. Furthermore, flavonoids have anticancer effects on breast tumors through multiple mechanisms (Martinez-Perez et al., 2014; Magne Nde et al., 2015; Zhang et al., 2018; Sudhakaran et al., 2019). Flavonoids can inhibit procarcinogen bioactivation and estrogen-producing and estrogen-metabolizing enzymes (Surichan et al., 2012; Miron et al., 2017), as well as breast cancer resistance protein (BCRP) (Fan et al., 2019). Administering flavonoids could inhibit inflammation, proliferation, tumor growth, and metastasis (Peluso et al., 2013; Khan et al., 2021; Guo et al., 2022). Although many studies have shown the pharmacological effects of flavonoids widely distributed in litchi seeds, almost all such studies were based on the extraction, enrichment, and separation of bioactive components, and few have focused on the spatial distribution and expression states of flavonoids. In fact, the precise reveal of the distribution of these flavonoids in litchi seeds is important for understanding the physiological and biochemical functions of these compounds and facilitating their extraction and utilization.
Matrix-assisted laser desorption/ionization mass spectrometry imaging (MALDI-MSI) has emerged as a molecular-imaging tool for simultaneously detecting and characterizing the spatial distribution and relative abundance of endogenous and exogenous compounds, such as lipids, proteins, metabolites, peptides, and drugs (Van De Plas et al., 2015; Qin et al., 2018; Piehowski et al., 2020). Although MALDI‐MSI has been used in plant science with endogenous molecular profiling to determine the spatial distribution of small molecules in plant tissues (Zaima et al., 2010; Taira et al., 2015; Huang et al., 2016; Li et al., 2018a), to the best of our knowledge, no previous study has utilized MALDI-MSI to characterize the spatial distribution of flavonoids in litchi seeds.
This study is the first to use MALDI-MSI for the in situ detection and imaging of flavonoids in litchi seed tissues. The results clearly showed the heterogeneous distribution of flavonoids in litchi seeds, indicating the potential of litchi seeds as a source for flavonoid extraction. MALDI-MS-based multi-imaging enhanced the visualization of spatial distribution and expression states of flavonoids. Our findings provide insights into the spatial distribution of flavonoids in litchi seeds and support the development of MALDI-MSI-based metabolomics as an appealing and credible molecular imaging technique for evaluating the spatial distribution of endogenous compounds.
Materials and methods
Materials and reagents
Fresh litchi fruit was collected from the Yongfuda litchi orchard (Haikou, Hainan, China) in June 2022. Haikou is located on Hainan Island in China. It has a typical tropical marine climate and annual sunshine duration of over 2,000 h. The climate is humid, the temperature rises fast, and the average annual precipitation is approximately 260 mm. The Yongfuda litchi orchard is located in a volcanic rock soil planting area. Once harvested, the peel and flesh of the litchi were immediately removed, and the litchi seeds were flash-frozen with liquid nitrogen by slow immersion to prevent seed shattering and endogenous compound changes. The commonly used MALDI matrix, 2-mercaptobenzothiazole (2-MBT), was obtained from Sigma-Aldrich (St. Louis, MO, USA). Amino acid and oligopeptide standards, including His, Gly-Gly-Leu (tripeptide), Ala-His-Lys (tripeptide), Leu-Leu-Tyr (tripeptide), and Arg-Gly-Asp-dTyr-Lys (pentapeptide), were purchased from Bankpeptide Biological Technology Co., Ltd. (Hefei, Anhui, China). Trifluoroacetic acid (TFA) and liquid chromatography–mass spectrometry (LC-MS)-grade methanol and ethanol were obtained from Merck & Co., Inc. (Darmstadt, Germany). Ultrapure water in the whole process of the experiments was prepared using a Millipore Milli-Q system (Bedford, MA, USA). All other reagents and chemicals were purchased from Merck, unless otherwise noted.
Tissue sectioning
For tissue sectioning, a Leica CM1860 cryostat (Leica Microsystems Inc., Wetzlar, Germany) was used. The frozen litchi seeds were cryo-sectioned into 12-μm-thick slices at a temperature of −20°C, and then the cryo-sectioned samples were thaw-mounted instantly on the conductive indium tin oxide films of microscope glass slides purchased from Bruker Daltonics (Bremen, Germany) (Figures 1A, B).
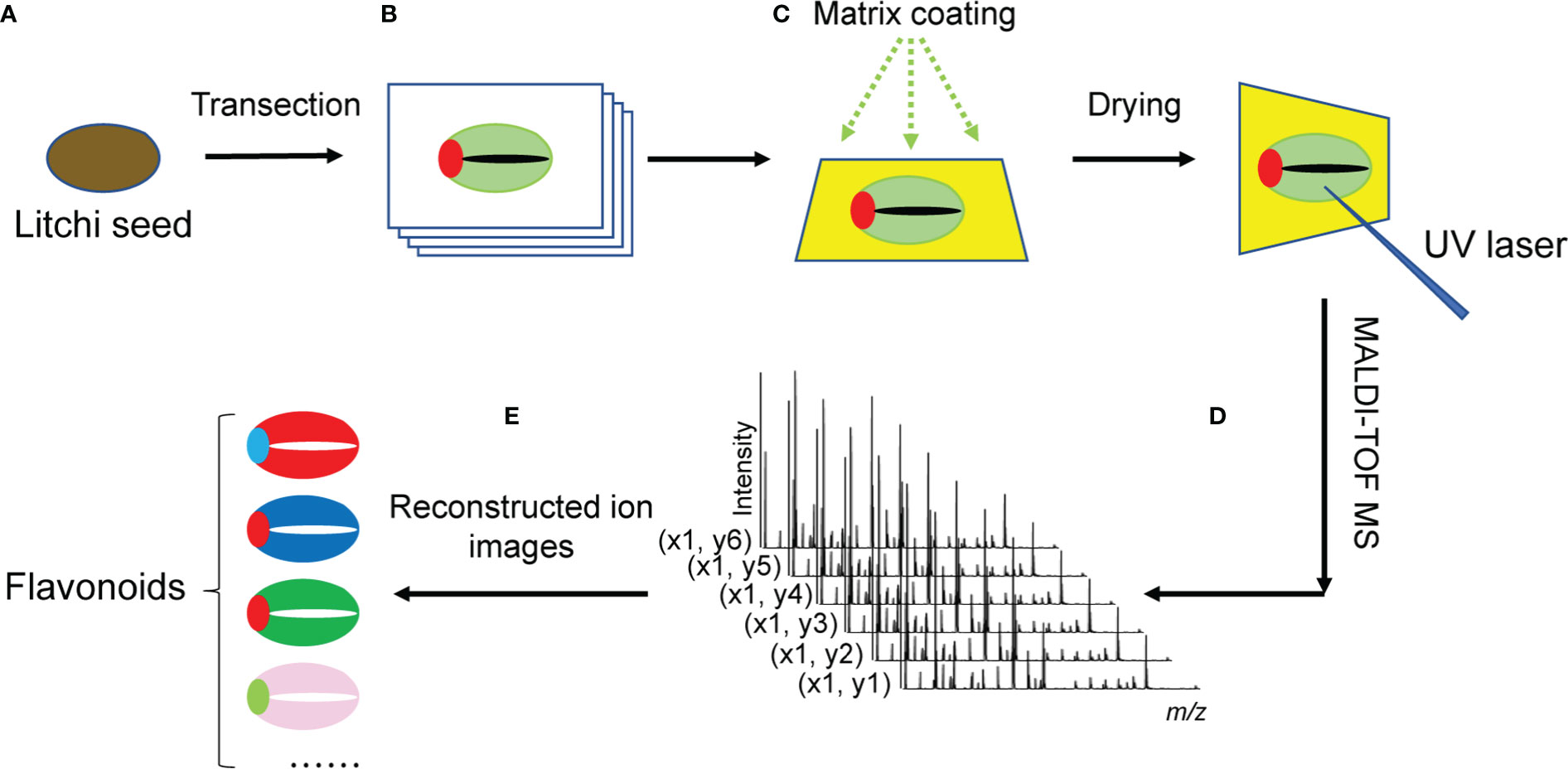
Figure 1 Schematic diagram of MALDI-MSI procedure for imaging flavonoids in litchi seeds. (A) Whole litchi seeds were used for transection into 12-μm-thick slices in a cryostat microtome. (B) Serial tissue sections were immediately thaw-mounted on the conductive sides of indium tin oxide (ITO)-coated microscope glass slides. Optical images of the litchi seed section were obtained using a scanner. (C) To assist ionization, the sections were coated with the organic matrix. (D) MALDI-TOF-MS was used to detect analytes in situ on the surface of litchi seed tissue sections. The mass spectra of ionized analytes were acquired at each detected pixel point. (E) MS images of analytes were reconstructed from the MS spectra obtained at each laser spot using specific imaging reconstruction software. MALDI-MSI, matrix-assisted laser desorption/ionization mass spectrometry imaging; TOF, time of fight; MS, mass spectrometry.
Matrix coating
After being air-dried, the serial litchi seed tissue sections were used for MALDI matrix coating (Figure 1C). A 2-MBT matrix solution was prepared at an optimal concentration of 15 mg/ml and dissolved in methanol/water/TFA (80:20:0.2, v/v/v). Air-dried tissue sections were coated with the 2-MBT matrix solution by a GET-Sprayer (III) (HIT Co., Ltd, Beijing, China). Briefly, the 2-MBT matrix solution 15 cycles (5 s spray, 10 s incubation, and 20 s drying time) was sprayed on the surface of the tissue sections to pre-seed a thin layer of the 2-MBT matrix. After the tissue sections were completely air-dried in a vented fume hood, the matrix solution was evenly sprayed for 50 more of the same cycles.
Histological staining
In order to obtain the histological images of litchi seed tissue sections, a slightly modified hematoxylin and eosin staining method was carried out based on an established procedure (Casadonte and Caprioli, 2011). Briefly, the tissue sections were washed in a series of ethanol solutions (100%, 95%, 80%, and 70% aqueous ethanol; 15 s/wash). After 10-s ultrapure water washing, tissue sections were stained with hematoxylin solution for 2 min and then washed with ultrapure water and 70% and 95% aqueous ethanol for 30 s each. The eosin solution was applied for another 1 min. Then, all tissue sections were washed with 95% and 100% ethanol and xylene for 30-s dehydration.
Optimal image acquisition
Optical images of the tissue sections were acquired using an Epson Perfection V550 photo scanner (Seiko Epson Corp, Suwa, Japan) according to previous studies (Wu et al., 2021; Shi et al., 2022).
MALDI-MS
An Autoflex Speed MALDI time-of-fight (TOF)/TOF mass spectrometer (Bruker Daltonics) with a MALDI source equipped with a 2,000-Hz solid-state Smartbeam Nd : YAG UV laser (355 nm, Azura Laser AG, Berlin, Germany) was used for profiling and imaging (Figure 1D).
To acquire in situ (+) MS profiling data of flavonoids from the tissue sections, all mass spectra were obtained over the m/z range of 100 to 700, each mass spectrum included an accumulation of 50 laser scans, and each scan was amassed from 500 laser shots. Three biological replicates of the sample and three technical replicates of each biological replicate were performed for MALDI-MS data acquisition (n = 3 × 3). To acquire the images of flavonoids, a 250-μm laser raster step-size was utilized for flavonoid in situ detection in tissues, and each pixel (scan spot) included 300 laser shots. With the use of FlexImaging 4.1 (Bruker Daltonics), the three “teaching points” for the correct positioning of the solid-state UV laser (Smartbeam Nd : YAG) for spectral acquisition were marked around a tissue section using a white ink correction pen. The m/z values of the compound ions that can be used for external mass calibration were listed as follows: His ([M+H]+, m/z 156.0768), Gly-Gly-Leu (tripeptide, [M+H]+, m/z 246.1448), Ala-His-Lys (tripeptide, [M+H]+, m/z 355.2088), Leu-Leu-Tyr (tripeptide, [M+H]+, m/z 408.2493), and Arg-Gly-Asp-dTyr-Lys (pentapeptide, [M+H]+, m/z 620.3151). Gly-Gly-Leu (tripeptide, [M+H]+, m/z 246.1448) and Arg-Gly-Asp-dTyr-Lys (pentapeptide, [M+H]+, m/z 620.3151) ions were selected in combination with the matrix ion of 2-MBT([M+H]+, m/z 167.9942) for internal mass calibration in the cubic enhanced mode. For the MALDI-TOF-MS analysis, MS/MS spectra were acquired in collision-induced dissociation (CID) mode, and argon was used as the collision gas. The flavonoid fragment ions were acquired under the following condition: ion source 1, 19.0 kV; ion source 2, 17.4 kV; lens, 8.8 kV; reflector 1, 21.0 kV; reflector 2, 9.8 kV; and accelerating voltage, 20.0 kV. The UV laser power ranged from 65% to 90%. MS/MS spectra were recorded based on no less than 5,000 laser shots over the m/z range of 0 to 100 with a sampling rate of 2.00 G/s, a detector gain of 9.5×, and an electronic gain of 100 mV.
Data analysis
For the MS profiling and MS/MS data analysis, Bruker FlexAnalysis 3.4 (Bruker Daltonics) was used for the preliminary viewing and processing of the mass spectra. Once the monoisotopic peak list was generated and exported, two metabolome databases (METLIN and HMDB) (Tautenhahn et al., 2012; Wishart et al., 2022) were used for the search of the detected m/z values of precursor ions and CID fragment ions against potential metabolite identities within an acceptable mass error of ±5 ppm. Three ion adduct forms (i.e., [M + H]+, [M + Na]+, and [M + K]+) were considered for the database search. For MALDI tissue imaging, Bruker FlexImaging 4.1 software was used for the reconstitution of the ion maps of the detected flavonoids (Figure 1E). For the generation of the ion images using FlexImaging, the mass filter width was set at 5 ppm.
Flavonoid extraction and identification by LC-MS/MS
Flavonoids were extracted from the seeds of litchi for LC-MS/MS analysis. The details of the extraction of the flavonoids from litchi seeds and the procedure of LC-MS/MS analysis for the identification and structural confirmation of the flavonoids can be found in the Supplementary Material.
Results and discussion
Morphological characteristics of litchi seeds
As shown in Figures 2A, B, under a light microscope, the litchi seed showed the following structures: testa, micropyle, embryo, cotyledon, and cotyledon gap. Among these structures, the testa was dark coffee-colored, the embryo was brown, and the cotyledon was oyster white. In addition, a gap was observed in the middle of the cotyledon. After hematoxylin and eosin staining, litchi seeds were observed again under a light microscope (Figure 2C). The anatomical structure of the litchi seeds is illustrated in Figure 2D.
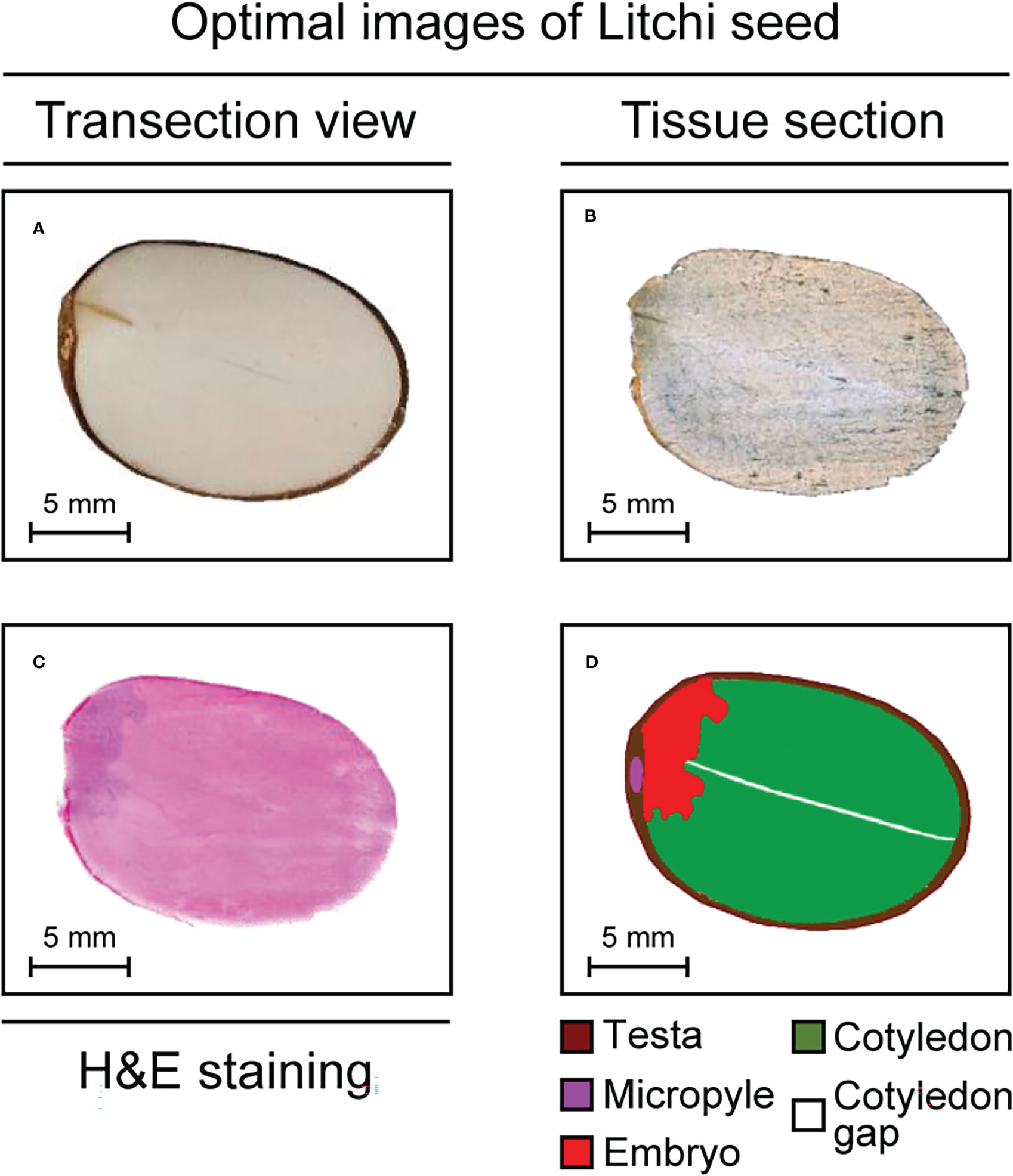
Figure 2 Optimal images of litchi seed tissue sections. (A, B) Photos of litchi seed tissue sections. (C) An H&E-stained litchi seed tissue section. (D) A cartoon of anatomical structure of litchi seed tissue section. H&E, hematoxylin and eosin.
Flavonoids detected in situ by MALDI-TOF-MS
As shown in Figure 3, many flavonoid-related signals were detected in the m/z range of 100–700. These compounds were confirmed by comparing the m/z values and MS/MS spectra with those obtained by LC-MS/MS (Table 1). According to collision-induced dissociation, 15 flavonoids compounds were identified through MALDI-TOF-MS: liquiritigenin (m/z 257.081, [M+H]+), apigenin (m/z 271.060, [M+H]+), naringenin (m/z 273.076, [M+H]+), daidzein (m/z 293.020, [M+Na]+), luteolin (m/z 287.056, [M+H]+), dihydrokaempferol (m/z 289.071, [M+H]+), catechin (m/z 329.043, [M+H]+), quercetin (m/z 303.051, [M+H]+), kaempferol (m/z 309.036, [M+Na]+), isorhamnetin (m/z 317.066, [M+H]+), myricetin (m/z 319.046, [M+H]+), quercetin 3-β-d-glucoside (m/z 465.102, [M+H]+), baicalin (m/z 469.073, [M+Na]+), rutin (m/z 649.118, [M+K]+), and taxifolin (m/z 305.065, [M+H]+).
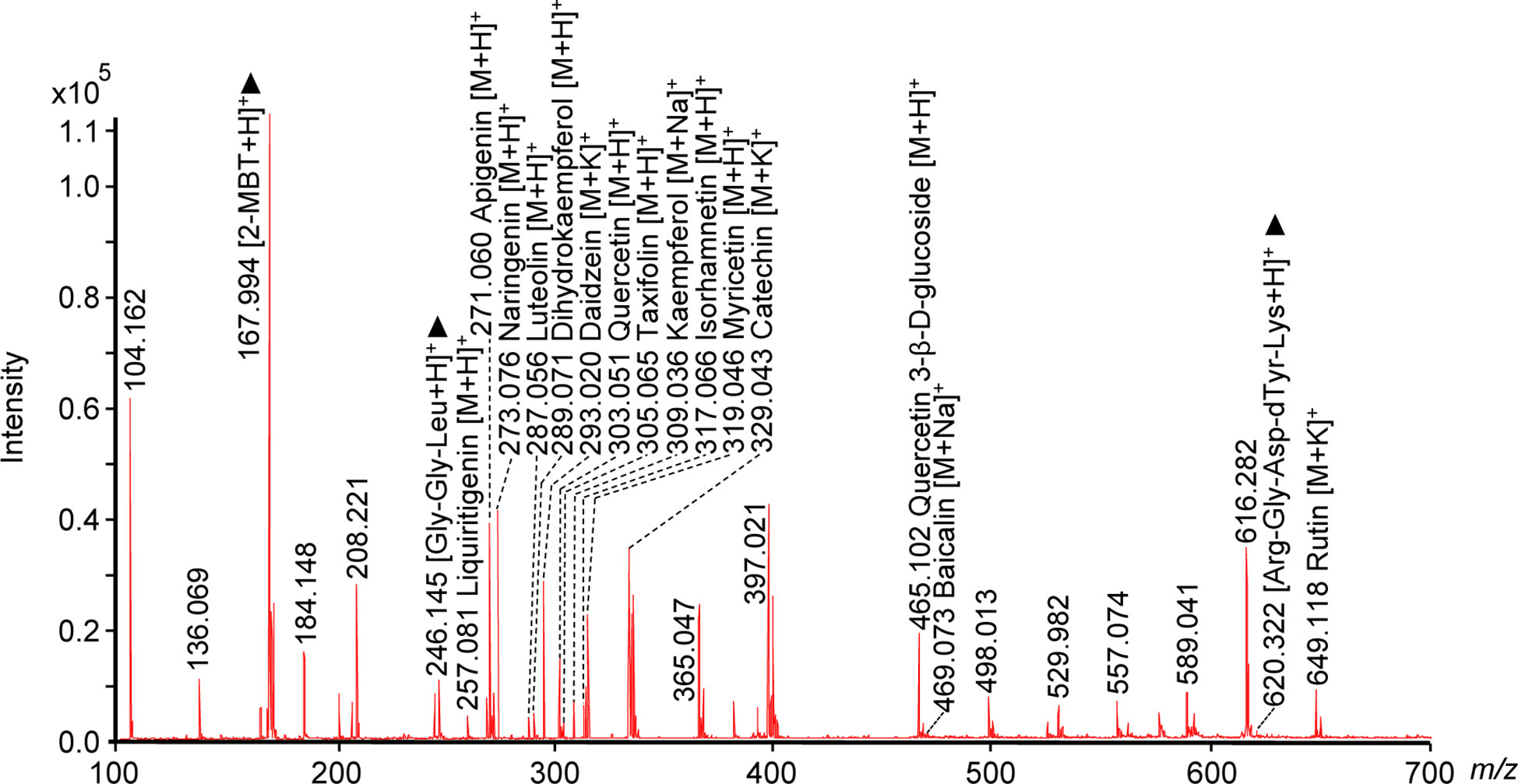
Figure 3 Mass spectrum of flavonoids detected in situ in a litchi seed tissue section using MALDI-TOF-MS and 2-MBT as the matrix in the positive ion mode. Two peptide standard ions, Gly-Gly-Leu (tripeptide, [M+H]+, m/z 246.145) and Arg-Gly-Asp-dTyr-Lys (pentapeptide, [M+H]+, m/z 620.322), and one matrix ion, 2-MBT ([M+H]+, m/z 167.994), were used as the reference peaks and are labeled with black triangle “▲”. Three biological repetitions and three technical repetitions were performed (n = 3 × 3). MALDI, matrix-assisted laser desorption/ionization; TOF, time-of-fight; MS, mass spectrometry; 2-MBT, 2-mercaptobenzothiazole.
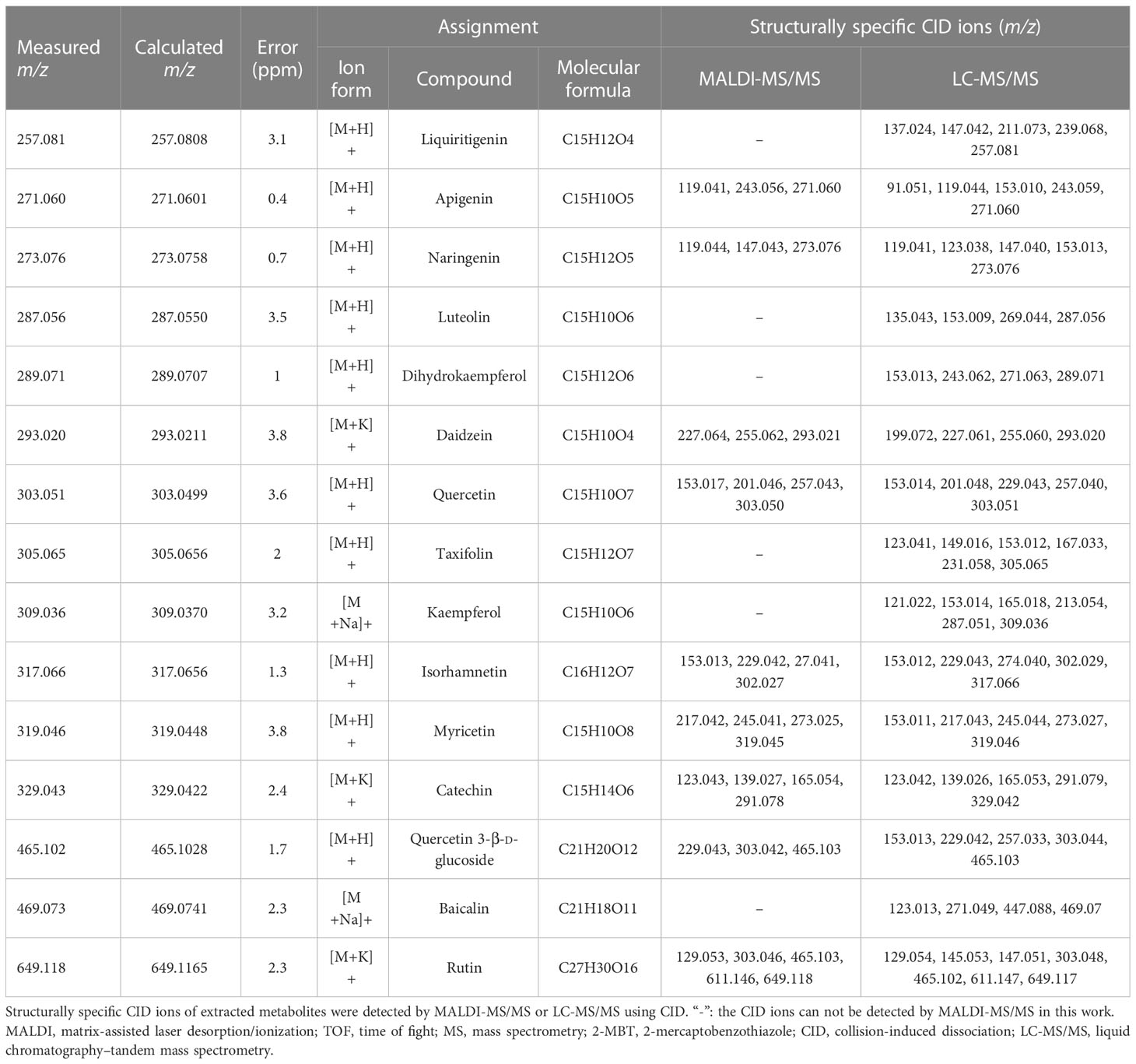
Table 1 The lists of 15 detectable flavonoids in litchi seed tissue sections by MALDI-TOF-MS using 2-MBT as the matrix in the positive ion mode.
MALDI-MS imaging of flavonoids
MALDI-MSI can provide a snapshot of the distribution of molecules at a specific location on a tissue surface. We present the mass spectrometry images of all 15 flavonoids and performed our classification analysis in Figure 4.
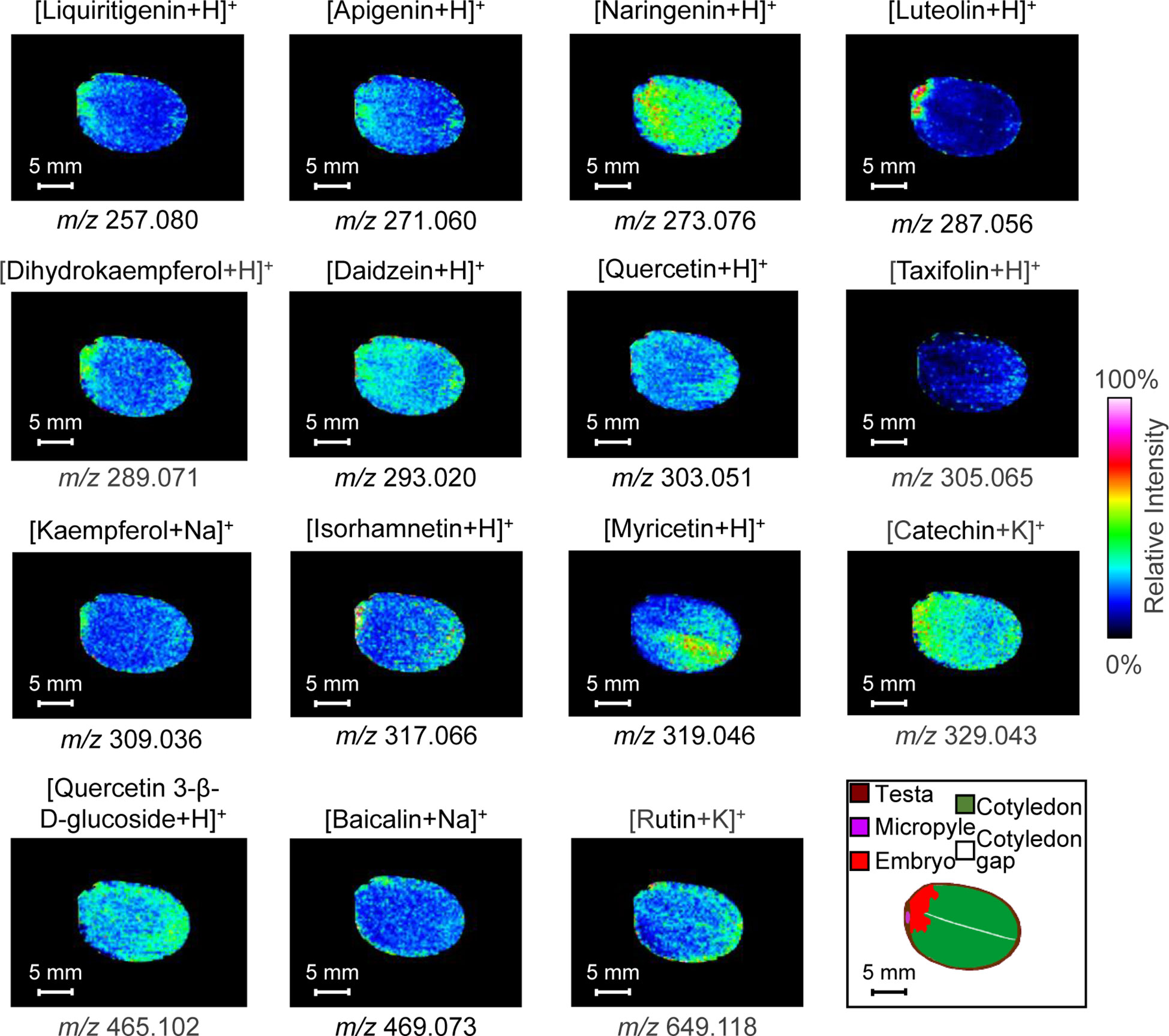
Figure 4 Ion images of 15 detectable flavonoids in litchi seed tissue sections from MALDI-TOF-MS using 2-MBT as the matrix in positive ion mode. MS imaging was acquired at 250-μm spatial resolution. MALDI, matrix-assisted laser desorption/ionization; TOF, time of fight; MS, mass spectrometry; 2-MBT, 2-mercaptobenzothiazole.
Ion images of the 15 flavonoids indicated that they can be broadly classified into four types. Four compounds were distributed mainly in the embryo: liquiritigenin (m/z 257.081, [M+H]+), luteolin (m/z 287.056, [M+H]+), dihydrokaempferol (m/z 289.071, [M+H]+), and kaempferol (m/z 309.036, [M+Na]+). Luteolin was highly concentrated in the embryo and less concentrated in other parts, while kaempferol was distributed at low abundance in the cotyledons and more in the embryo. Myricetin (m/z 319.046, [M+H]+), baicalin (m/z 469.073, [M+Na]+), and rutin (m/z 649.118, [M+K]+) were primarily distributed in the cotyledons. Baicalin and rutin were distributed at the periphery of the cotyledons, and myricetin was distributed to one side of the cotyledon gap. Most of the compounds were distributed in both cotyledons and embryos, including naringenin (m/z 273.076, [M+H]+), apigenin (m/z 271.060, [M+H]+), daidzein (m/z 293.020, [M+Na]+), quercetin (m/z 303.051, [M+H]+), isorhamnetin (m/z 317.066, [M+H]+), catechin (m/z 329.043, [M+H]+), and quercetin 3-β-d-glucoside (m/z 465.102, [M+H]+). Naringenin and catechin were concentrated throughout the litchi seed, their distribution being more homogeneous and without obvious tissue specificity. Quercetin, quercetin 3-β-d-glucoside, and apigenin were distributed at the periphery of the cotyledons and in the embryo. The compound daidzein was uniformly distributed, whereas isorhamnetin was more distributed at the apical part of the cotyledons. Finally, the taxifolin (m/z 305.065, [M+H]+) content was low and mainly distributed in the inner seed testa.
Four compounds were mainly distributed in the embryo: liquiritigenin, luteolin, dihydrokaempferol, and kaempferol. As the embryo is the most important part of the seed in plant development, these flavonoids may provide essential substances for growth and development and improve seed resistance. Luteolin was highly concentrated in the embryo and less concentrated in other parts. Luteolin, through inducing root nodulation, plays an important role in nitrogen metabolism in nitrogen-fixing plants and enhanced plant stress tolerance by promoting its nitrogen enrichment (Peters et al., 1986). Liquiritigenin was also mainly concentrated in the embryo and lesser in the cotyledons close to the embryo. Liquiritigenin increases ultraviolet irradiation, indicating its anti-radiation function (Sun et al., 2012). Dihydrokaempferol and kaempferol were interconvertible; therefore, both had similar distribution characteristics and are distributed in the cotyledons as well as the embryo. Many studies have demonstrated that kaempferol, as a precursor of ubiquitin-ketone (coenzyme Q) biosynthesis, is an atypical node between primary and specialized metabolism (Soubeyrand et al., 2018; Berger et al., 2022). Kaempferol is involved in plant defense and signaling in response to stressful conditions (Soubeyrand et al., 2018; Jan et al., 2022). Dihydrokaempferol is involved in plant growth and development. As a precursor of orange pelargonidin-type anthocyanins, dihydrokaempferol plays a role in flower coloring (Johnson et al., 2001). Liquiritigenin rapidly inactivates the PI3K/AKT/mTOR pathway. In vivo studies demonstrated that liquiritigenin can significantly inhibit tumor growth, increase cell autophagy, and accelerate cell apoptosis. In addition, it attenuates the malignant-like biological behaviors in triple-negative breast cancer cells through its induction of autophagy-related apoptosis via the PI3K/AKT/mTOR pathway (Ji et al., 2021), decreased DNMT activity, and elevated BRCA1 expression and transcriptional activity (Liang et al., 2021). Dihydrokaempferol has strong anti-inflammatory and antioxidant activities, which can improve the inflammatory performance and oxidative stress state of acute pancreatitis (Liang et al., 2020; Zhang et al., 2021). In contrast, kaempferol shows more pharmacological activities, such as anti-bacterial (Yeon et al., 2019), anti-inflammatory (Yeon et al., 2019), anti-oxidant (Chen and Chen, 2013), antitumor (Calderón-Montaño et al., 2011), and anti-diabetic activities (Yang et al., 2021b), and are cardio-protective (Chen et al., 2022b) and neuro-protective (Wang et al., 2020). Currently, kaempferol is also commonly used in cancer chemotherapy (Ren et al., 2019). The mechanisms of kaempferol’s anticancer include apoptosis, cell cycle arrest at the G2/M phase, downregulation of epithelial–mesenchymal transition-related markers, and repression of overactivation of the phosphatidylinositol 3-kinase/protein kinase B signaling pathway (Imran et al., 2019; Wang et al., 2019). Luteolin sensitizes cancer cells to treatment-induced cytotoxicity via suppressing cell survival pathways and enhancing apoptosis pathways, including the apoptosis pathway of the tumor suppressor protein p53 (Lin et al., 2008). These compounds can be extracted from the embryo of litchi seeds, which is convenient for obtaining a higher content of target substances for pharmaceutical and mass production in the future.
Myricetin, baicalin, and rutin were mainly found in the cotyledons of litchi seeds. Myricetin was mainly concentrated on one side of the cotyledon gap, while rutin and baicalin were mainly distributed at the periphery (Figure 4). From a physiological point of view, flavonoids such as myricetin and baicalin assist in the reinforcement of plant tissues, maintenance of seed dormancy, and longevity of seeds during storage (Shirley, 1998). Rutin may participate in strengthening the plant’s defense system against environmental stresses, including UV exposure, low-temperature stress, drought stress, and bacterial pathogen infection (Suzuki et al., 2015; Yang et al., 2016). Myricetin has therapeutic effects on a variety of diseases, such as inflammation, cerebral ischemia, Alzheimer’s disease (AD), cancer, diabetes, pathogenic microorganism infection, thrombosis, and atherosclerosis (Song et al., 2021). Furthermore, myricetin has been reported to regulate the expression of STAT3, PI3K/AKT/mTOR, AChE, IκB/NF-κB, BrdU/NeuN, Hippo, eNOS/NO, ACE, MAPK, Nrf2/HO-1, TLR, and GSK-3β (Song et al., 2021). Rutin shows clear antioxidant and anticancer effects, including a strong ability to inhibit tumors in breast cancer, especially triple-negative breast cancer (Iriti et al., 2017; Liang et al., 2021). Baicalin, similar to rutin and myricetin, has inhibitory effects on lung, breast, and bladder cancers, through different signaling pathways and mechanisms (Ge et al., 2021; Kong et al., 2021; Zhao et al., 2021). Owing to their important pharmacological effects, our study of their spatial distribution provided a basis for the precise extraction of flavonoids for developing drugs.
Seven flavonoids, i.e., naringenin, apigenin, daidzein, quercetin, isorhamnetin, catechin, and quercetin-3-β-d-glucoside, were mainly found in both the cotyledon and embryo of litchi seeds. Among these compounds, catechin, naringenin, daidzein, apigenin, and quercetin-3-β-d-glucoside have homogeneous distributions with relatively high abundance. Isorhamnetin was mainly distributed in the radicle and tip of the cotyledon, while quercetin was distributed at the periphery of the cotyledon. Flavonoids are secondary metabolites in plants that play a critical role in impairing ultraviolet irradiation, regulating the oxidative stress response, and influencing the transport of plant hormones, flower coloring, and pathogen resistance (Buer et al., 2010; Chen et al., 2022a). Naringenin plays various roles in plant–microbe interactions (An et al., 2021). Lignin biosynthesis and coenzyme ligase (4CL) are involved in plant growth, and naringenin is one of the metabolites in this pathway that inhibit enzymes such as 4-CL (Deng et al., 2004). Apigenin (4′,5,7-trihydroxyflavone) is a bioactive compound that belongs to the flavone class, and it is the aglycone of many naturally occurring glycosides. It ameliorates the damaging effects of salinity on rice seedlings, presumably by regulating selective ion uptake by roots and translocation to shoots, thus maintaining the higher K+/Na+ ratio critical for normal plant growth under salinity stress (Mekawy et al., 2018). Daidzein, as an isoflavonoid, plays crucial roles in the expression of the nod genes of rhizobial bacteria. The expression of this compound in roots will increase the synthesis and secretion of nodulation factors, promoting a series of physiological changes in plant cells and initiating the formation of nodules (Bosse et al., 2021). Quercetin promotes a series of physiological and biochemical processes in plants, including seed germination, pollen growth, photosynthesis, and antioxidant machinery, thus facilitating proper plant growth and development (Singh et al., 2021). In addition, quercetin is an antioxidant that enhances plant resistance to some biotic and abiotic stresses. Quercetin-3-β-d-glucoside is a quercetin-derived compound with attached glucose instead of the 3-OH group of quercetin. Isorhamnetin is a methylated flavonoid derived from quercetin. Catechins, as a type of flavonoid, also belong to phenolic compounds. Making up more than 70% of polyphenols, catechins consist of ester and non-ester catechins. The multifunctional catechins contribute to decreased reactive oxygen species and better adaptability of plants to the environment (Jiang et al., 2020). Some of these flavonoids have been previously extracted from litchi seeds, for example, catechin and naringenin (Zhu et al., 2019). Similar to other flavonoids, most of these compounds have many pharmacological effects, including anti-inflammatory, antioxidant, and antidiabetic activities. In particular, since the start of the COVID-19 epidemic, antiviral activity has been reported for catechin (Mishra et al., 2021) and quercetin (Bernini and Velotti, 2021). The antitumor effects of flavonoids have also been extensively studied, with the following mechanisms reported: inducing oxidative stress (Souza et al., 2017), enhancing chemotherapy drug effect (Yang et al., 2021a), and regulating signaling pathways (Amado et al., 2014). Notably, daidzein is a phytohormone similar to estrogens and thus may have a therapeutic effect on estrogen-dependent diseases (Meng et al., 2017). Therefore, flavonoid compounds are useful for developing drug-based therapies, and exploring the distribution of flavonoids will facilitate efficient extraction and utilization.
Although taxifolin was successfully detected in sections in situ using MALDI-MSI, the abundance of this compound was low. As shown in Figure 4, taxifolin was mainly found in the testa and peripheral part of the cotyledons, indicating that the compound can protect seed embryos from external biotic and abiotic factors, such as soil microbes (e.g., fungi and bacteria) and saline-alkali abiotic stress, thus improving seed vitality and germination rate (Ninfali et al., 2020; Wan et al., 2020). By regulating the aromatic hydrocarbon receptor/cytochrome P450 1A1 (CYP1A1) signaling pathway, taxifolin can significantly inhibit the proliferation, migration, invasion, and viability of gastric cancer cells (Xie et al., 2021). Similarly, the same effect of taxifolin has been observed on breast cancer by promoting mesenchymal-to-epithelial transition (EMT) through β-catenin signaling (Von Minckwitz et al., 2019).
Conclusion
MALDI-MSI was used for in situ detection and imaging of flavonoid distribution in litchi seeds for the first time. Overall, 15 flavonoids were successfully imaged. Among them, four (dihydrokaempferol, liquiritigenin, luteolin, and kaempferol) were distributed in the seed embryo, three (rutin, baicalin, and myricetin) were mainly found in the cotyledons, seven (quercetin, naringenin, isorhamnetin, daidzein, apigenin, catechin, and quercetin 3-β-d-glucoside) were enriched in both the embryo and cotyledons, and one (taxifolin) was mainly detected in the inner testa. Our MALDI-MSI results showed clear tissue distribution heterogeneity for the different flavonoid compounds in litchi seeds. Such information will be important for further study to understand the physiological and chemical functions of such flavonoid compounds. Furthermore, our study provides a basis for further improving the efficiency of extracting and utilizing bioactive compounds from litchi seeds.
Data availability statement
The original contributions presented in the study are included in the article/Supplementary Material. Further inquiries can be directed to the corresponding author.
Author contributions
Concepts: YL and XN. Design: ZW, FJ, and YL. Literature search: JW, ZZ, and YL. Data acquisition and analysis: ZW, XN, YL, and ZZ. Writing—original draft: YL, XN, and JW. Writing—review and editing: FJ, ZW, YL, and XN. Funding acquisition: YL, XN, and JW. Supervision: FJ and ZW. All authors contributed to the article and approved the submitted version.
Funding
This work was supported by grants from the fund of Qingdao Medical and Health Research Project (grant number: 2021-WJZD090).
Conflict of interest
The authors declare that the research was conducted in the absence of any commercial or financial relationships that could be construed as a potential conflict of interest.
Publisher’s note
All claims expressed in this article are solely those of the authors and do not necessarily represent those of their affiliated organizations, or those of the publisher, the editors and the reviewers. Any product that may be evaluated in this article, or claim that may be made by its manufacturer, is not guaranteed or endorsed by the publisher.
Supplementary material
The Supplementary Material for this article can be found online at: https://www.frontiersin.org/articles/10.3389/fpls.2023.1144449/full#supplementary-material
References
Amado, N. G., Predes, D., Fonseca, B. F., Cerqueira, D. M., Reis, A. H., Dudenhoeffer, A. C., et al. (2014). Isoquercitrin suppresses colon cancer cell growth in vitro by targeting the wnt/β-catenin signaling pathway. J. Biol. Chem. 289, 35456–35467. doi: 10.1074/jbc.M114.621599
An, J., Kim, S. H., Bahk, S., Vuong, U. T., Nguyen, N. T., Do, H. L., et al. (2021). Naringenin induces pathogen resistance against pseudomonas syringae through the activation of NPR1 in arabidopsis. Front. Plant Sci. 12. doi: 10.3389/fpls.2021.672552
Berger, A., Latimer, S., Stutts, L. R., Soubeyrand, E., Block, A. K., Basset, G. J. (2022). Kaempferol as a precursor for ubiquinone (coenzyme q) biosynthesis: An atypical node between specialized metabolism and primary metabolism. Curr. Opin. Plant Biol. 66, 102165. doi: 10.1016/j.pbi.2021.102165
Berk, Ş., Kaya, S., Akkol, E. K., Bardakçı, H. (2022). A comprehensive and current review on the role of flavonoids in lung cancer-experimental and theoretical approaches. Phytomedicine 98, 153938. doi: 10.1016/j.phymed.2022.153938
Bernini, R., Velotti, F. (2021). Natural polyphenols as immunomodulators to rescue immune response homeostasis: Quercetin as a research model against severe COVID-19. Molecules 26. doi: 10.3390/molecules26195803
Bosse, M. A., Silva, M. B. D., Oliveira, N., Araujo, M. A., Rodrigues, C., Azevedo, J. P., et al. (2021). Physiological impact of flavonoids on nodulation and ureide metabolism in legume plants. Plant Physiol. Biochem. 166, 512–521. doi: 10.1016/j.plaphy.2021.06.007
Buer, C. S., Imin, N., Djordjevic, M. A. (2010). Flavonoids: New roles for old molecules. J. Integr. Plant Biol. 52, 98–111. doi: 10.1111/j.1744-7909.2010.00905.x
Calderón-Montaño, J. M., Burgos-Morón, E., Pérez-Guerrero, C., López-Lázaro, M. (2011). A review on the dietary flavonoid kaempferol. Mini Rev. Med. Chem. 11, 298–344. doi: 10.2174/138955711795305335
Casadonte, R., Caprioli, R.M. (2011). Proteomic analysis of formalin-fixed paraffin-embedded tissue by MALDI imaging mass spectrometry. Nat. Protoc. 6, 1695–1709. doi: 10.1038/nprot.2011.388
Chen, A. Y., Chen, Y. C. (2013). A review of the dietary flavonoid, kaempferol on human health and cancer chemoprevention. Food Chem. 138, 2099–2107. doi: 10.1016/j.foodchem.2012.11.139
Chen, J. H., Hou, N., Xv, X., Zhang, D., Fan, T. Q., Zhang, Q. X., et al. (2022a). Flavonoid synthesis and metabolism during the fruit development in hickory (Carya cathayensis). Front. Plant Sci. 13. doi: 10.3389/fpls.2022.896421
Chen, M., Xiao, J., El-Seedi, H. R., Woźniak, K. S., Daglia, M., Little, P. J., et al. (2022b). Kaempferol and atherosclerosis: From mechanism to medicine. Crit. Rev. Food Sci. Nutr. 1-19. doi: 10.1080/10408398.2022.2121261
Choi, S.-A., Lee, J. E., Kyung, M. J., Youn, J. H., Oh, J. B., Whang, W. K. (2017). Anti-diabetic functional food with wasted litchi seed and standard of quality control. Appl. Biol. Chem. 60, 197–204. doi: 10.1007/s13765-017-0269-9
Ciumărnean, L., Milaciu, M. V., Runcan, O., Vesa Ș, C., Răchișan, A. L., Negrean, V., et al. (2020). The effects of flavonoids in cardiovascular diseases. Molecules 25. doi: 10.3390/molecules25184320
Deng, F., Aoki, M., Yogo, Y. (2004). Effect of naringenin on the growth and lignin biosynthesis of gramineous plants. Weed Biol. Manage. 4, 49–55. doi: 10.1111/j.1445-6664.2003.00119.x
Dong, X., Huang, Y., Wang, Y., He, X. (2019). Anti-inflammatory and antioxidant jasmonates and flavonoids from lychee seeds. J. Funct. Foods 54, 74–80. doi: 10.1016/j.jff.2018.12.040
Emanuele, S., Lauricella, M., Calvaruso, G., D'anneo, A., Giuliano, M. (2017). Litchi chinensis as a functional food and a source of antitumor compounds: An overview and a description of biochemical pathways. Nutrients 9, 992. doi: 10.3390/nu9090992
Fan, X., Bai, J., Zhao, S., Hu, M., Sun, Y., Wang, B., et al. (2019). Evaluation of inhibitory effects of flavonoids on breast cancer resistance protein (BCRP): From library screening to biological evaluation to structure-activity relationship. Toxicol. In Vitro 61, 104642. doi: 10.1016/j.tiv.2019.104642
Ge, A., Liu, L., Deng, X., Luo, J., Xu, Y. (2021). Exploring the mechanism of baicalin intervention in breast cancer based on MicroRNA microarrays and bioinformatics strategies. Evid. Based Complement. Alternat. Med. 2021, 7624415. doi: 10.1155/2021/7624415
Guo, Y., Ding, S. J., Ding, X., Liu, Z., Wang, J. L., Chen, Y., et al. (2022). Effects of selected flavonoids oncellproliferation and differentiation of porcine muscle stem cells for cultured meat production. Food Res. Int. 160, 111459. doi: 10.1016/j.foodres.2022.111459
Hu, F.-C., Chen, Z., Wang, X.-H., Wang, J.-B., Fan, H.-Y., Qin, Y.-H., et al. (2021). Construction of high-density SNP genetic maps and QTL mapping for dwarf-related traits in litchi chinensis sonn. J. Integr. Agr. 20, 2900–2913. doi: 10.1016/s2095-3119(20)63387-1
Huang, L., Tang, X., Zhang, W., Jiang, R., Chen, D., Zhang, J., et al. (2016). Imaging of endogenous metabolites of plant leaves by mass spectrometry based on laser activated electron tunneling. Sci. Rep. 6, 24164. doi: 10.1038/srep24164
Imran, M., Salehi, B., Sharifi-Rad, J., Aslam Gondal, T., Saeed, F., Imran, A., et al. (2019). Kaempferol: A key emphasis to its anticancer potential. Molecules 24. doi: 10.3390/molecules24122277
Iriti, M., Kubina, R., Cochis, A., Sorrentino, R., Varoni, E. M., Kabała-Dzik, A., et al. (2017). Rutin, a quercetin glycoside, restores chemosensitivity in human breast cancer cells. Phytother. Res. 31, 1529–1538. doi: 10.1002/ptr.5878
Jan, R., Khan, M., Asaf, S., Lubna, Asif, S., Kim, K. M. (2022). Bioactivity and therapeutic potential of kaempferol and quercetin: New insights for plant and human health. Plants (Basel) 11. doi: 10.3390/plants11192623
Ji, Y., Hu, W., Jin, Y., Yu, H., Fang, J. (2021). Liquiritigenin exerts the anti-cancer role in oral cancer via inducing autophagy-related apoptosis through PI3K/AKT/mTOR pathway inhibition in vitro and in vivo. Bioengineered 12, 6070–6082. doi: 10.1080/21655979.2021.1971501
Jiang, C. K., Ma, J. Q., Liu, Y. F., Chen, J. D., Ni, D. J., Chen, L. (2020). Identification and distribution of a single nucleotide polymorphism responsible for the catechin content in tea plants. Hortic. Res. 7, 24. doi: 10.1038/s41438-020-0247-y
Johnson, E. T., Ryu, S., Yi, H., Shin, B., Cheong, H., Choi, G. (2001). Alteration of a single amino acid changes the substrate specificity of dihydroflavonol 4-reductase. Plant J. 25, 325–333. doi: 10.1046/j.1365-313x.2001.00962.x
Khan, H., Ullah, H., Martorell, M., Valdes, S. E., Belwal, T., Tejada, S., et al. (2021). Flavonoids nanoparticles in cancer: Treatment, prevention and clinical prospects. Semin. Cancer Biol. 69, 200–211. doi: 10.1016/j.semcancer.2019.07.023
Kong, N., Chen, X., Feng, J., Duan, T., Liu, S., Sun, X., et al. (2021). Baicalin induces ferroptosis in bladder cancer cells by downregulating FTH1. Acta Pharm. Sin. B. 11, 4045–4054. doi: 10.1016/j.apsb.2021.03.036
Li, H., Huang, D., Ma, Q., Qi, W., Li, H. (2020). Factors influencing the technology adoption behaviours of litchi farmers in China. Sustainability 12, 271. doi: 10.3390/su12010271
Li, B., Neumann, E. K., Ge, J., Gao, W., Yang, H., Li, P., et al. (2018a). Interrogation of spatial metabolome of ginkgo biloba with high-resolution matrix-assisted laser desorption/ionization and laser desorption/ionization mass spectrometry imaging. Plant Cell Environ. 41, 2693–2703. doi: 10.1111/pce.13395
Li, Y., Zhang, T., Chen, G. Y. (2018b). Flavonoids and colorectal cancer prevention. Antioxidants (Basel) 7. doi: 10.3390/antiox7120187
Liang, X., Hu, C., Liu, C., Yu, K., Zhang, J., Jia, Y. (2020). Dihydrokaempferol (DHK) ameliorates severe acute pancreatitis (SAP) via Keap1/Nrf2 pathway. Life Sci. 261, 118340. doi: 10.1016/j.lfs.2020.118340
Liang, F., Zhang, H., Gao, H., Cheng, D., Zhang, N., Du, J., et al. (2021). Liquiritigenin decreases tumorigenesis by inhibiting DNMT activity and increasing BRCA1 transcriptional activity in triple-negative breast cancer. Exp. Biol. Med. (Maywood) 246, 459–466. doi: 10.1177/1535370220957255
Lin, Y., Shi, R., Wang, X., Shen, H. M. (2008). Luteolin, a flavonoid with potential for cancer prevention and therapy. Curr. Cancer Drug Targets 8, 634–646. doi: 10.2174/156800908786241050
Liskova, A., Samec, M., Koklesova, L., Brockmueller, A., Zhai, K., Abdellatif, B., et al. (2021). Flavonoids as an effective sensitizer for anti-cancer therapy: Insights into multi-faceted mechanisms and applicability towards individualized patient profiles. Epma. J. 12, 155–176. doi: 10.1007/s13167-021-00242-5
Magne Nde, C. B., Zingue, S., Winter, E., Creczynski-Pasa, T. B., Michel, T., Fernandez, X., et al. (2015). Flavonoids, breast cancer chemopreventive and/or chemotherapeutic agents. Curr. Med. Chem. 22, 3434–3446. doi: 10.2174/0929867322666150729115321
Maleki, S. J., Crespo, J. F., Cabanillas, B. (2019). Anti-inflammatory effects of flavonoids. Food Chem. 299, 125124. doi: 10.1016/j.foodchem.2019.125124
Martinez-Perez, C., Ward, C., Cook, G., Mullen, P., Mcphail, D., Harrison, D. J., et al. (2014). Novel flavonoids as anti-cancer agents: mechanisms of action and promise for their potential application in breast cancer. Biochem. Soc. Trans. 42, 1017–1023. doi: 10.1042/BST20140073
Mekawy, A. M. M., Abdelaziz, M. N., Ueda, A. (2018). Apigenin pretreatment enhances growth and salinity tolerance of rice seedlings. Plant Physiol. Biochem. 130, 94–104. doi: 10.1016/j.plaphy.2018.06.036
Meng, H., Fu, G., Shen, J., Shen, K., Xu, Z., Wang, Y., et al. (2017). Ameliorative effect of daidzein on cisplatin-induced nephrotoxicity in mice via modulation of inflammation, oxidative stress, and cell death. Oxid. Med. Cell. Longev. 2017, 3140680. doi: 10.1155/2017/3140680
Miron, A., Aprotosoaie, A. C., Trifan, A., Xiao, J. (2017). Flavonoids as modulators of metabolic enzymes and drug transporters. Ann. N. Y. Acad. Sci. 1398, 152–167. doi: 10.1111/nyas.13384
Mishra, C. B., Pandey, P., Sharma, R. D., Malik, M. Z., Mongre, R. K., Lynn, A. M., et al. (2021). Identifying the natural polyphenol catechin as a multi-targeted agent against SARS-CoV-2 for the plausible therapy of COVID-19: An integrated computational approach. Brief Bioinform. 22, 1346–1360. doi: 10.1093/bib/bbaa378
Ninfali, P., Panato, A., Bortolotti, F., Valentini, L., Gobbi, P. (2020). Morphological analysis of the seeds of three pseudocereals by using light microscopy and ESEM-EDS. Eur. J. Histochem. 64. doi: 10.4081/ejh.2020.3075
Pan, H., Zhou, W., He, W., Liu, X., Ding, Q., Ling, L., et al. (2012). Genistein inhibits MDA-MB-231 triple-negative breast cancer cell growth by inhibiting NF-kappaB activity via the notch-1 pathway. Int. J. Mol. Med. 30, 337–343. doi: 10.3892/ijmm.2012.990
Park, K. I., Park, H. S., Nagappan, A., Hong, G. E., Lee, D. H., Kang, S. R., et al. (2012). Induction of the cell cycle arrest and apoptosis by flavonoids isolated from Korean citrus aurantium l. @ in non-small-cell lung cancer cells. Food Chem. 135, 2728–2735. doi: 10.1016/j.foodchem.2012.06.097
Peluso, I., Raguzzini, A., Serafini, M. (2013). Effect of flavonoids on circulating levels of TNF-alpha and IL-6 in humans: a systematic review and meta-analysis. Mol. Nutr. Food Res. 57, 784–801. doi: 10.1002/mnfr.201200721
Peters, N. K., Frost, J. W., Long, S. R. (1986). A plant flavone, luteolin, induces expression of rhizobium meliloti nodulation genes. Science 233, 977–980. doi: 10.1126/science.3738520
Piehowski, P. D., Zhu, Y., Bramer, L. M., Stratton, K. G., Zhao, R., Orton, D. J., et al. (2020). Automated mass spectrometry imaging of over 2000 proteins from tissue sections at 100-mum spatial resolution. Nat. Commun. 11, 8. doi: 10.1038/s41467-019-13858-z
Punia, S., Kumar, M. (2021). Litchi (Litchi chinenis) seed: Nutritional profile, bioactivities, and its industrial applications. Trends Food Sci. Technol. 108, 58–70. doi: 10.1016/j.tifs.2020.12.005
Qin, L., Zhang, Y., Liu, Y., He, H., Han, M., Li, Y., et al. (2018). Recent advances in matrix-assisted laser desorption/ionisation mass spectrometry imaging (MALDI-MSI) for in situ analysis of endogenous molecules in plants. Phytochem. Anal. 29, 351–364. doi: 10.1002/pca.2759
Rakha, A., Umar, N., Rabail, R., Butt, M. S., Kieliszek, M., Hassoun, A., et al. (2022). Anti-inflammatory and anti-allergic potential of dietary flavonoids: A review. Biomed. Pharmacother. 156, 113945. doi: 10.1016/j.biopha.2022.113945
Ren, J., Lu, Y., Qian, Y., Chen, B., Wu, T., Ji, G. (2019). Recent progress regarding kaempferol for the treatment of various diseases (Review). Exp. Ther. Med. 18, 2759–2776. doi: 10.3892/etm.2019.7886
Shi, Y., Hu, H., Hao, Q., Wu, R., Wang, L., Qin, L., et al. (2022). Michler's ethylketone as a novel negative-ion matrix for the enhancement of lipid MALDI tissue imaging. Chem. Commun. (Camb.) 58, 633–636. doi: 10.1039/d1cc05718a
Shirley, B. W. (1998). Flavonoids in seeds and grains: Physiological function, agronomic importance and the genetics of biosynthesis. Seed Sci. Res. 8, 415–422. doi: 10.1017/s0960258500004372
Singh, P., Arif, Y., Bajguz, A., Hayat, S. (2021). The role of quercetin in plants. Plant Physiol. Biochem. 166, 10–19. doi: 10.1016/j.plaphy.2021.05.023
Song, X., Tan, L., Wang, M., Ren, C., Guo, C., Yang, B., et al. (2021). Myricetin: A review of the most recent research. Biomed. Pharmacother. 134, 111017. doi: 10.1016/j.biopha.2020.111017
Soubeyrand, E., Johnson, T. S., Latimer, S., Block, A., Kim, J., Colquhoun, T. A., et al. (2018). The peroxidative cleavage of kaempferol contributes to the biosynthesis of the benzenoid moiety of ubiquinone in plants. Plant Cell 30, 2910–2921. doi: 10.1105/tpc.18.00688
Souza, R. P., Bonfim-Mendonca, P. S., Gimenes, F., Ratti, B. A., Kaplum, V., Bruschi, M. L., et al. (2017). Oxidative stress triggered by apigenin induces apoptosis in a comprehensive panel of human cervical cancer-derived cell lines. Oxid. Med. Cell. Longev. 2017, 1512745. doi: 10.1155/2017/1512745
Sudhakaran, M., Sardesai, S., Doseff, A. I. (2019). Flavonoids: New frontier for immuno-regulation and breast cancer control. Antioxidants (Basel) 8. doi: 10.3390/antiox8040103
Sun, R., Hikosaka, S., Goto, E., Sawada, H., Saito, T., Kudo, T., et al. (2012). Effects of UV irradiation on plant growth and concentrations of four medicinal ingredients in Chinese licorice (Glycyrrhiza uralensis). Acta Hortic. 956, 643–648. doi: 10.17660/ActaHortic.2012.956.77
Surichan, S., Androutsopoulos, V. P., Sifakis, S., Koutala, E., Tsatsakis, A., Arroo, R. R., et al. (2012). Bioactivation of the citrus flavonoid nobiletin by CYP1 enzymes in MCF7 breast adenocarcinoma cells. Food Chem. Toxicol. 50, 3320–3328. doi: 10.1016/j.fct.2012.06.030
Suzuki, T., Morishita, T., Kim, S.-J., Park, S.-U., Woo, S.-H., Noda, T., et al. (2015). Physiological roles of rutin in the buckwheat plant. Japan Agric. Res. Quarterly: JARQ 49, 37–43. doi: 10.6090/jarq.49.37
Taira, S., Tokai, M., Kaneko, D., Katano, H., Kawamura-Konishi, Y. (2015). Mass spectrometry imaging analysis of location of procymidone in cucumber samples. J. Agric. Food Chem. 63, 6109–6112. doi: 10.1021/acs.jafc.5b00957
Tang, Y., Yu, C., Wu, J., Chen, H., Zeng, Y., Wang, X., et al. (2018). Lychee seed extract protects against neuronal injury and improves cognitive function in rats with type II diabetes mellitus with cognitive impairment. Int. J. Mol. Med. 41, 251–263. doi: 10.3892/ijmm.2017.3245
Tautenhahn, R., Cho, K., Uritboonthai, W., Zhu, Z., Patti, G. J., Siuzdak, G. (2012). An accelerated workflow for untargeted metabolomics using the METLIN database. Nat. Biotechnol. 30, 826–828. doi: 10.1038/nbt.2348
Van De Plas, R., Yang, J., Spraggins, J., Caprioli, R. M. (2015). Image fusion of mass spectrometry and microscopy: A multimodality paradigm for molecular tissue mapping. Nat. Methods 12, 366–372. doi: 10.1038/nmeth.3296
Von Minckwitz, G., Huang, C. S., Mano, M. S., Loibl, S., Mamounas, E. P., Untch, M., et al. (2019). Trastuzumab emtansine for residual invasive HER2-positive breast cancer. N. Engl. J. Med. 380, 617–628. doi: 10.1056/NEJMoa1814017
Vue, B., Zhang, S., Chen, Q. H. (2016). Flavonoids with therapeutic potential in prostate cancer. Anticancer Agents Med. Chem. 16, 1205–1229. doi: 10.2174/1871520615666151008122622
Wan, L., Lei, Y., Yan, L., Liu, Y., Pandey, M. K., Wan, X., et al. (2020). Transcriptome and metabolome reveal redirection of flavonoids in a white testa peanut mutant. BMC Plant Biol. 20, 161. doi: 10.1186/s12870-020-02383-7
Wang, J., Mao, J., Wang, R., Li, S., Wu, B., Yuan, Y. (2020). Kaempferol protects against cerebral ischemia reperfusion injury through intervening oxidative and inflammatory stress induced apoptosis. Front. Pharmacol. 11. doi: 10.3389/fphar.2020.00424
Wang, X., Yang, Y., An, Y., Fang, G. (2019). The mechanism of anticancer action and potential clinical use of kaempferol in the treatment of breast cancer. Biomed. Pharmacother. 117, 109086. doi: 10.1016/j.biopha.2019.109086
Wishart, D. S., Guo, A., Oler, E., Wang, F., Anjum, A., Peters, H., et al. (2022). HMDB 5.0: The human metabolome database for 2022. Nucleic Acids Res. 50, D622–D631. doi: 10.1093/nar/gkab1062
Wu, R., Qin, L., Chen, L., Ma, R., Chen, D., Liu, H., et al. (2021). Copper adhesive tape attached to the reverse side of a non-conductive glass slide to achieve protein MALDI-imaging in FFPE-tissue sections. Chem. Commun. (Camb.) 57, 10707–10710. doi: 10.1039/d1cc03629g
Xie, J., Pang, Y., Wu, X. (2021). Taxifolin suppresses the malignant progression of gastric cancer by regulating the AhR/CYP1A1 signaling pathway. Int. J. Mol. Med. 48. doi: 10.3892/ijmm.2021.5030
Yang, L., Gao, Y., Bajpai, V. K., El-Kammar, H. A., Simal-Gandara, J., Cao, H., et al. (2021b). Advance toward isolation, extraction, metabolism and health benefits of kaempferol, a major dietary flavonoid with future perspectives. Crit. Rev. Food Sci. Nutr. 1–17. doi: 10.1080/10408398.2021.1980762
Yang, C., Song, J., Hwang, S., Choi, J., Song, G., Lim, W. (2021a). Apigenin enhances apoptosis induction by 5-fluorouracil through regulation of thymidylate synthase in colorectal cancer cells. Redox Biol. 47, 102144. doi: 10.1016/j.redox.2021.102144
Yang, W., Xu, X., Li, Y., Wang, Y., Li, M., Wang, Y., et al. (2016). Rutin-mediated priming of plant resistance to three bacterial pathogens initiating the early SA signal pathway. PLos One 11, e0146910. doi: 10.1371/journal.pone.0146910
Yao, P., Gao, Y., Simal-Gandara, J., Farag, M. A., Chen, W., Yao, D., et al. (2021). Litchi (Litchi chinensis sonn.): A comprehensive review of phytochemistry, medicinal properties, and product development. Food Funct. 12, 9527–9548. doi: 10.1039/d1fo01148k
Yeon, M. J., Lee, M. H., Kim, D. H., Yang, J. Y., Woo, H. J., Kwon, H. J., et al. (2019). Anti-inflammatory effects of kaempferol on helicobacter pylori-induced inflammation. Biosci. Biotechnol. Biochem. 83, 166–173. doi: 10.1080/09168451.2018.1528140
Zaima, N., Goto-Inoue, N., Hayasaka, T., Setou, M. (2010). Application of imaging mass spectrometry for the analysis of oryza sativa rice. Rapid Commun. Mass Spectrom. 24, 2723–2729. doi: 10.1002/rcm.4693
Zhang, H. W., Hu, J. J., Fu, R. Q., Liu, X., Zhang, Y. H., Li, J., et al. (2018). Flavonoids inhibit cell proliferation and induce apoptosis and autophagy through downregulation of PI3Kγ mediated PI3K/AKT/mTOR/p70S6K/ULK signaling pathway in human breast cancer cells. Sci. Rep. 8, 11255. doi: 10.1038/s41598-018-29308-7
Zhang, J., Hu, C., Li, X., Liang, L., Zhang, M., Chen, B., et al. (2021). Protective effect of dihydrokaempferol on acetaminophen-induced liver injury by activating the SIRT1 pathway. Am. J. Chin. Med. 49, 705–718. doi: 10.1142/S0192415X21500324
Zhao, L., Wang, K., Wang, K., Zhu, J., Hu, Z. (2020). Nutrient components, health benefits, and safety of litchi (Litchi chinensis sonn.): A review. Compr. Rev. Food Sci. Food Saf. 19, 2139–2163. doi: 10.1111/1541-4337.12590
Zhao, F., Zhao, Z., Han, Y., Li, S., Liu, C., Jia, K. (2021). Baicalin suppresses lung cancer growth phenotypes via miR-340-5p/NET1 axis. Bioengineered 12, 1699–1707. doi: 10.1080/21655979.2021.1922052
Keywords: litchi seed, flavonoid, matrix-assisted laser desorption/ionization, mass spectrometry imaging, spatial distribution
Citation: Liu Y, Nie X, Wang J, Zhao Z, Wang Z and Ju F (2023) Visualizing the distribution of flavonoids in litchi (Litchi chinenis) seeds through matrix-assisted laser desorption/ionization mass spectrometry imaging. Front. Plant Sci. 14:1144449. doi: 10.3389/fpls.2023.1144449
Received: 14 January 2023; Accepted: 13 February 2023;
Published: 24 February 2023.
Edited by:
Xiaodong Wang, Minzu University of China, ChinaCopyright © 2023 Liu, Nie, Wang, Zhao, Wang and Ju. This is an open-access article distributed under the terms of the Creative Commons Attribution License (CC BY). The use, distribution or reproduction in other forums is permitted, provided the original author(s) and the copyright owner(s) are credited and that the original publication in this journal is cited, in accordance with accepted academic practice. No use, distribution or reproduction is permitted which does not comply with these terms.
*Correspondence: Fang Ju, MjkxODM4Njc5QHFxLmNvbQ==
†These authors have contributed equally to this work