- 1Department of Plant Protection, Faculty of Agriculture Akdeniz University, Antalya, Türkiye
- 2Department of Entomology, Directorate of Plant Protection Research Institute, Bornova, İzmir, Türkiye
- 3Biosciences, University of Exeter, Exeter, United Kingdom
- 4Department of Biological Sciences, School of Science and the Environment, University of Worcester, Worcester, United Kingdom
Root-knot nematodes (RKNs, Meloidogyne spp.) can cause severe yield losses in tomatoes. The Mi-1.2 gene in tomato confers resistance to the Meloidogyne species M. incognita, M. arenaria and M. javanica, which are prevalent in tomato growing areas. However, this resistance breaks down at high soil temperatures (>28°C). Therefore, it is imperative that new resistance sources are identified and incorporated into commercial breeding programmes. We identified a tomato line, MT12, that does not have Mi-1.2 but provides resistance to M. incognita at 32°C soil temperature. An F2 mapping population was generated by crossing the resistant line with a susceptible line, MT17; the segregation ratio showed that the resistance is conferred by a single dominant gene, designated RRKN1 (Resistance to Root-Knot Nematode 1). The RRKN1 gene was mapped using 111 Kompetitive Allele Specific PCR (KASP) markers and characterized. Linkage analysis showed that RRKN1 is located on chromosome 6 and flanking markers placed the locus within a 270 kb interval. These newly developed markers can help pyramiding R-genes and generating new tomato varieties resistant to RKNs at high soil temperatures.
Introduction
Tomato (Solanum lycopersicum) is an economically important vegetable crop grown in regions with warm and temperate climates. Root-knot nematodes (RKNs) are one of the most important pests of tomato plants and are obligate endoparasites that can cause galls on the roots of the plant they feed on and restrict the uptake of water and nutrients from the vascular bundles. Therefore, they cause a decrease in yield and quality (Williamson and Hussey, 1996).
Management methods for RKNs include crop rotation with resistant varieties or less- susceptible crops (Talavera et al., 2009; Abd-Elgawad, 2022), cultural and tillage practices (Marquez and Hajihassani, 2023), microbial biocontrol agents (Hashem and Abo-Elyousr, 2011) and nematicides (Rawal, 2020; Phani et al., 2021). Using resistant varieties is one of the most common and successful control methods today due to their lack of residue, ease of application and environmental friendliness (Devran and Söğüt, 2010). Plant disease resistance genes have been classified into eight major families based on their amino acid motif organization and their membrane spanning domains (Gururani et al., 2012). The majority of resistance genes encode proteins belonging to the toll/interleukin1 receptor (TIR) – nucleotide binding site – leucine-rich repeat (TIR-NBS-LRR) or intracellular coiled coil (CC) –nucleotide binding site – leucine-rich repeat (CC-NBS-LRR, Mchale et al., 2006), which are now called NLRs (Barragan and Weigel, 2021). The Mi-1.2 gene encodes a CC-NLR protein (Milligan et al., 1998) and provides effective protection against Meloidogyne incognita, M. javanica and M. arenaria, which are commonly present in tomato growing areas (Roberts and Thomason, 1986). However, the Mi-1.2 gene loses its effect at high soil temperatures and the resistance breaks down (Dropkin, 1969; Özalp and Devran, 2018). Especially in hot climates, the increase in soil temperatures in summer plantings creates problems for growers using tomato varieties carrying the Mi-1.2 gene. This climate-derived condition severely limits the use of resistant tomato cultivars carrying the Mi-1.2 gene. Therefore, it is imperative to identify new resistance gene(s) for the sustainability of resistance against RKN. Gene(s) conferring resistance at high soil temperature may allow earlier planting and prolong the growing period in hot climates regions. Therefore, researchers have identified new resistance genes from Mi-2 to Mi-9. Among these genes, Mi-2, Mi-3, Mi-4, Mi-5, Mi-6 and Mi-9 were found to be effective at high soil temperatures (Cap et al., 1993; Yaghoobi et al., 1995; Veremis and Roberts, 1996a; Veremis and Roberts, 1996b; Veremis et al., 1999; Ammiraju et al., 2003). Of these genes, Mi-2 and Mi-4 have not yet been mapped; Mi-3 and Mi-5 have been mapped to chromosome 12 (Yaghoobi et al., 1995; Ammiraju et al., 2003; Jablonska et al., 2007), while Mi-1, Mi-6 and Mi-9 have been mapped to chromosome 6 (Veremis and Roberts, 1996b; Kaloshian et al., 1998; Milligan et al., 1998; Ammiraju et al., 2003; Jablonska et al., 2007). Gene Mi-3 additionally provides resistance against Mi-1.2-virulent RKN populations (Yaghoobi et al., 1995), while Mi-6 and Mi-9 are susceptible to Mi-1.2-virulent RKN populations (Veremis and Roberts, 1996b; Veremis and Roberts, 2000). Apart from these genes, Mi-HT provides durable resistance at high temperatures and shows homology to Mi-1.2 and Mi-9 (Wu et al., 2009). However, it is not known whether Mi-HT is a different gene from Mi-9 (Wang et al., 2013). The Mi-9 gene was recently characterized and cloned by Jiang et al. (2023) using a chromosome-scale genome assembly of Solanum arcanum LA2157. Seven candidate genes were determined using comparative genomic studies and markers shown to be linked to the Mi-9 gene. Transcriptomics demonstrated that five of the seven candidate genes were expressed in root tissues. Cloning of the Mi-9 gene was confirmed by silencing candidate genes and investigating the resistance performance of plants obtained. In addition, Mi-9 was transformed into susceptible tomato lines and was demonstrated to confirm resistance to RKN at high soil temperature (Jiang et al., 2023). Previously, we investigated the response of the Mi-1.2 gene at high soil temperatures and observed that the resistance provided by the Mi-1.2 gene broke down in plants exposed to 32°C soil temperature for 48 hours or longer (Özalp and Devran, 2018). We then proceeded to investigate the response of the identified tomato line along with the other tomato lines at high soil temperature. In this study, we determined that the tomato line MT12 confers resistance to the RKN, and the durability provided was heat stable. Thus, we focused our further studies on this line. We used next generation sequencing (NGS) technology to map this new gene in MT12 designated RRKN1 (Resistance to Root-Knot Nematode 1), and identified the genomic interval on the chromosome 6 for the RRKN1 using Kompetitive Allele Specific PCR (KASP) markers.
Materials and methods
Plant materials
The parental genotypes MT12 and MT17 were obtained from tomato genetic resources of Multi Tohum (Antalya, Turkey). The tomato line MT12 was used as a resistance source in this study. There is not much information about the genetic background of this genotype. MT17 is a susceptible parent obtained from female (Solanum lycopersicum X S. hirsutum) and male (Solanum lycopersicum X Solanum pimpinellifolium). Tomato cultivars Seval F1 carrying Mi-1.2 gene and susceptible Tueza F1 (Multi Tohum, Antalya, Turkey) were used as controls in the experiments. The presence or absence of Mi-1.2 gene in all tomato plants was confirmed using Mi23 primer set (Seah et al., 2007) (Supplementary Figure 1).
Nematode isolates
Two avirulent isolates of M. incognita, S6 and K7, were used in the experiment to investigate the response of the MT12 at a high temperature (Devran and Sogut, 2009). All isolates used have been continued as pure nematode cultures since 2008 in Devran laboratory. The responses of MT12 to Mi-1.2 natural virulent populations V12 and V21 of M. incognita were also investigated. Only the M. incognita avirulent S6 isolate was used in the mapping and inheritance studies of RRKN1.
Avirulent isolates were multiplied on susceptible tomato cultivar Tueza F1, while Mi-1.2 natural virulent isolates were multiplied on resistant tomato cultivar Seval F1 containing the Mi-1.2 gene to maintain their virulence in a growth chamber at 25°C ± 0.5 with a 16:8 hours photoperiod (light: dark) and 65 ± 5% relative humidity. Sixty days after inoculation the plants were removed, and the plant roots were washed with water. Egg masses were collected from the root using a small needle and placed in centrifuge tubes (Özalp and Devran, 2018). They were then stored at 4°C until inoculation.
Testing plant lines with nematode
Tomato seeds were sown in vials including vermiculite, perlite, and peat (v:v:v: 1:1:1) and maintained in a seedling facility. Seedlings with four true leaves were transplanted into 250 ml plastic pots including sterilized sandy soil and were kept in the growth chamber at 25°C for a week to ensure root development. For the tests to be carried out at high temperature, the pots were transferred to a growth chamber at 32°C. Soil temperature was monitored with the probes placed in pots and nematodes were inoculated when the soil temperature reached 32°C. Seedlings were exposed to soil temperatures at 32°C for 7 days.
For nematode inoculation, egg masses were placed in a sieve and fresh second stage juveniles (J2s) from hatched eggs were collected and counted under a light microscope and diluted to 1000 J2s/ml. A total of 1000 J2s were inoculated into two holes (0.5 ml of water per hole) near the stem of each plant. The procedure was performed according to former studies (Öçal et al., 2018; Nas et al., 2023). After seven days, the plants were transferred to a growth chamber at 25°C ± 0.5 with a 16:8 hours photoperiod (light: dark) and 65 ± 5% relative humidity until the end of the experiment.
Investigating MT12 response to avirulent and Mi-1.2 natural virulent isolates at high soil temperature
To observe the response of the MT12 tomato line to nematode isolates at a high temperature, an experiment was conducted under 25°C and 32°C soil temperature conditions. For this purpose, avirulent M. incognita isolates K7 and S6 were used for the inoculation of plants. Besides resistant source MT12, susceptible tomato cultivar Tueza F1 and resistant tomato cultivar Seval F1 were used as control plants in the experiment.
To observe the response of the MT12 tomato cultivar to Mi-1.2 natural virulent M. incognita isolates, an experiment was conducted under 25°C soil temperature conditions. For this purpose, Mi-1.2 natural virulent M. incognita isolates V12 and V21 were used for the inoculation of plants.
Both experiments were performed as described above for testing plant lines. The experiments were carried out as five replicates and two repeats according to the completely randomized design.
Inheritance and mapping population
A cross between the resistant and susceptible parent lines MT12 and MT17, respectively, was generated and the F1 line was obtained. An F2 mapping population was generated by selfing the F1 line. A total of 130 F2 seeds were obtained from a single F1 tomato plant and were used in the phenotyping and genotyping experiments.
Data evaluation and statistics
Plants were uprooted sixty days post inoculation (dpi) and the roots were washed under the tap water. The roots were then stained with Ploxine B, and the galls and egg masses were counted and recorded (Öçal et al., 2018). Egg masses and galls in the roots of the plants were counted under a light microscope.
Plants in mapping population were classified as resistant if the individual root system had less than 25 egg masses, or susceptible if the individual root system had 25 or more egg masses (Veremis et al., 1999; Ammiraju et al., 2003; Wang et al., 2013).
Data were analyzed by analysis of variance (ANOVA). Differences among means were examined using the Tukey multiple comparison test. Chi-square tests for specific proportions for goodness of fit were carried out using the PROC FREQ function. All statistical analysis was performed using with the SAS statistical program (v. 9.0 for Windows; SAS Institute Inc., Cary, NC, USA).
DNA isolation
Genomic DNA was extracted from young leaves collected from parental lines and F2 plants using the Wizard Magnetic Kit (Promega) following the manufacturer’s instructions. DNA was then run on an agarose gel and checked for degradation or their high molecular weight. The quality control (QC) of the DNA was determined by Novogene UK (Cambridge, United Kingdom) before proceeding to the sequencing.
Genome sequencing
The sequencing has been carried out by Novogene UK (Cambridge, United Kingdom), generating 150 bp paired-end read data for each parent line (resistant and susceptible) with NovaSeq 6000.
Bioinformatics and NGS analysis
As previously described (Kahveci et al., 2021), we took the NGS analysis approach and trimmed the raw reads using BBDuk (filterk=27, trimk=27; https://sourceforge.net/projects/bbmap/) to remove Illumina adapters and to quality-trim both ends to Q12. Trimmed sequences from parental lines were then mapped onto chromosomes 1 – 12 of the SL3.1 version of the available reference tomato genome (S. lycopersicum cultivar Heinz 1706, GenBank: GCA_000188115.4) using BBMap (https://sourceforge.net/projects/bbmap/) and the alignment data were converted to the BAM format (Li et al., 2009). The variant detection was performed using BCFtools (Li et al., 2009) and SNPsFromPileups (https://github.com/davidjstudholme/SNPsFromPileups) as previously described (Devran et al., 2018; Kahveci et al., 2021). Integrative Genomics Viewer (IGV) was used to visualize the alignment results (Robinson et al., 2011). We used the Solanaceae Genomics Network web portal (Fernandez-Pozo et al., 2015) to browse and visualize the tomato genomic interval and identify the genes contained therein.
Strategies for mapping the RRKN1 gene
At the beginning of the study, there was no information on the location of the RRKN1 gene. Therefore, we used three different strategies to determine the RRKN1 locus.
Strategy 1: focusing on chromosome 6
Previous studies reported that some genes providing resistance to RKNs in tomatoes are located on chromosome 6 (Milligan et al., 1998; Jablonska et al., 2007; Du et al., 2020). Therefore, initially, we focused on this chromosome. We selected 55 SNPs from the SolCap Tomato Genotyping Panel (https://www.biosearchtech.com) on chromosome 6, nine of which were polymorphic between parental lines (Supplementary Table 1).
Strategy 2: global mapping
Analysis of the genomic sequences of our parental lines identified 34 new SNPs, distributed over 10 chromosomes (Supplementary Table 2). Single-nucleotide differences among parents were visually inspected using IGV. In determining the number of primers to represent each chromosome, we considered the length of each chromosome in the reference genome of the tomato.
Strategy 3: information-led mapping
Since the data obtained from the first and second strategies provided information that the gene is most likely to be located on chromosome 6, we focused on this chromosome. Thus, genome sequences belonging to the parents were compared with the reference genome in the IGV and 17 new further SNPs were taken towards marker development and fine mapping (Supplementary Table 2).
For all three strategies, initially identified SNPs were used to detect polymorphisms between parental lines and then polymorphic markers were used to screen mapping population.
Development of KASP markers for RRKN1 gene
Genomic sequences flanking and including SNPs were determined using Geneious Prime 2019 (Biomatters) and sent to LGC Biosearch Technologies (https://www.biosearchtech.com) to develop KASP primers. The KASP reactions were performed according to previous studies (Devran and Kahveci, 2019; Kahveci et al., 2021) and carried out using the Hydrocycler (LGC Biosearch Technologies, UK) with a 61-55°C touchdown protocol. The fluorescent readings were performed for 2 min at 25°C using a FluOstar Omega Microplate Reader (BMG LABTECH Ortenberg, Germany).
Accession numbers
The raw sequence reads of parent lines have been deposited in the Sequence Read Archive (SRA) under accession numbers SRR25056976 and SRR25056975 and are accessible via BioProject accession PRJNA988534.
Results
Tomato line MT12 does not carry Mi-1.2 but provides resistance to Mi-1.2-avirulent isolates at high soil temperature
In this experiment, we investigated changes in the resistance status of tomato plants inoculated with M. incognita K7 and S6 isolates when exposed to soil temperatures of 25°C and 32°C. MT12 (mi-1.2/mi1.2) and Seval (Mi-1.2/mi-1.2) F1 plants were resistant to both isolates at 25°C soil temperature, whilst the Tueza (mi1.2/mi-1.2) F1 cultivar was susceptible, exhibiting a large number of galls and egg masses, as expected (see Supplementary Figure 1 for genotypes of plant lines used). No significant differences were observed in the gall and egg mass numbers of both isolates on MT12 and Seval F1 cultivar when exposed to 25°C soil temperature (p ≤ 0.05) (Table 1). Among the plants exposed to 32°C soil temperature, the highest egg mass and gall number was counted in Tueza F1. Resistance was broken at 32°C in Seval F1 carrying the Mi-1.2 gene; however, the gall and egg mass numbers were about half of those observed in the susceptible Tueza F1. Conversely, MT12 showed a resistant response against K7 and S6 isolates despite being exposed to 32°C soil temperature for one week (Table 1).
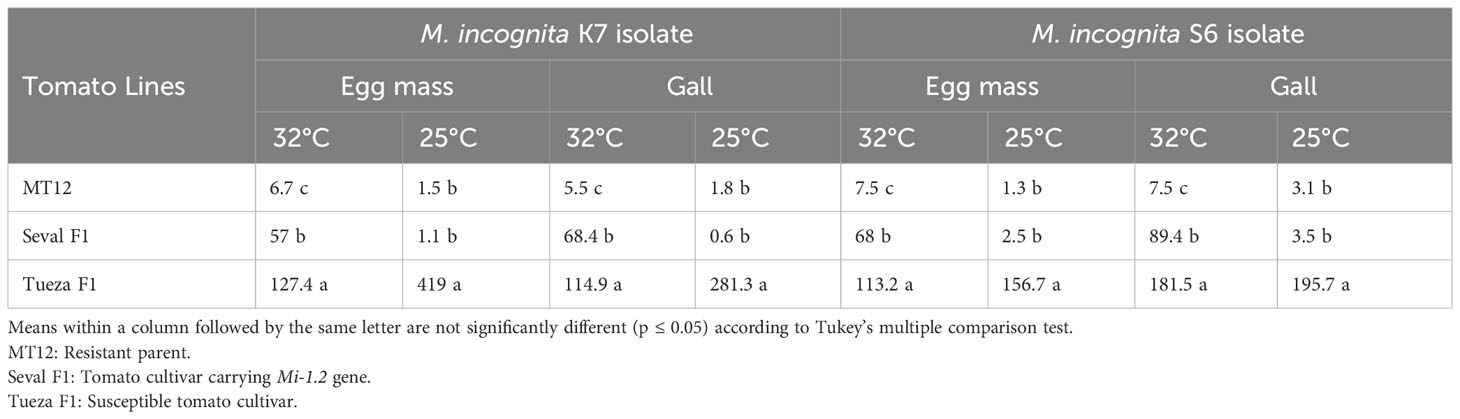
Table 1 Number of egg masses and galls of Meloidogyne incognita isolates on tomato cultivars exposed to 32°C and 25°C.
Tomato line MT12 is susceptible to naturally Mi-1.2-virulent isolates
We also tested MT12 with nematode isolates that are naturally Mi-1.2-virulent at 25˚C soil temperature. The Mi-1.2 naturally virulent isolates V12 and V21 multiplied very well and produced egg masses on the roots of MT12, indicating that MT12 did not provide resistance to Mi-1.2 naturally virulent isolates. Also, Seval F1 lines bearing the Mi-1.2 gene were overcome by the Mi-1.2-virulent isolates as expected. The isolates produced egg masses on roots of susceptible Tueza F1 and caused galls. No statistically significant differences were observed in the numbers of galls between the two virulent isolates in the three tested plant lines (p ≤ 0.05) (Table 2).
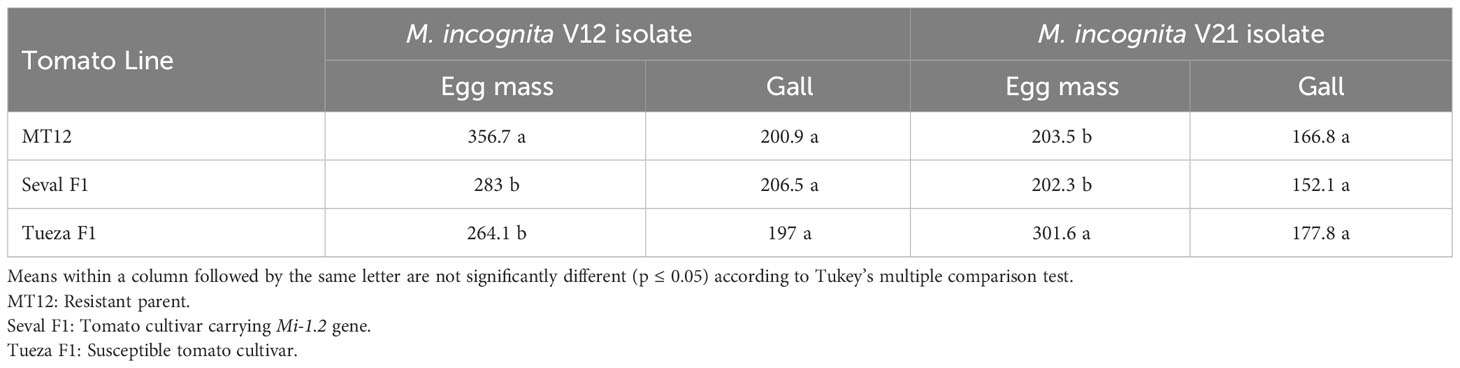
Table 2 Number of egg masses and galls of Mi-1.2-natural virulent Meloidogyne incognita isolates on tomato cultivars.
Resistance to M. incognita is controlled by a single dominant gene
We tested the parents and F1 plants derived from the cross between the susceptible and resistant tomato lines for resistance to M. incognita at 32°C soil temperature. The F1 individuals were resistant to M. incognita. The F1 plant was selfed to obtain F2 population. Of 130 F2 individuals subjected to M. incognita, screening showed 91 resistant and 39 susceptible plants, giving a segregation ratio of 3:1 resistant:susceptible (χ2 = 1,73, p=0.18). This indicates that the resistance is controlled by a single dominant gene designated Resistant to Root-Knot Nematode 1 (RRKN1) at 32°C soil temperature.
Mapping RRKN1 gene
Initially 55 SNPs on chromosome 6 of the tomato genome from SolCap were selected and converted to KASP markers. We first screened parents with these markers for polymorphism and 9 of them were found to be polymorphic (Supplementary Table 1). Then, F2 populations were screened with these polymorphic markers. Phenotype and genotype data indicated that RRKN1 may be located on chromosome 6.
We also took a global genome mapping approach. We developed KASP markers for all chromosomes, except for 8 and 9, for which no SNPs were identified. Markers (Supplementary Tables 2, 3) were first used to screen parents to confirm polymorphism and the F2 mapping population was screened. Results showed that only those KASP markers developed from chromosome 6 were linked to the pathological data for RRKN1. These findings supported our initial results where we used 9 KASP markers developed from SolCap. Therefore, we focused on chromosome 6 to confirm the location of RRKN1 gene and developed further new 17 KASP markers from chromosome 6 (Supplementary Table 2) to fine map the gene. Two flanking markers, KASP-6-2649872 and KASP-6-2919895, were identified placing the gene(s) in a 270 kb interval on chromosome 6 (Table 3; Figure 1).
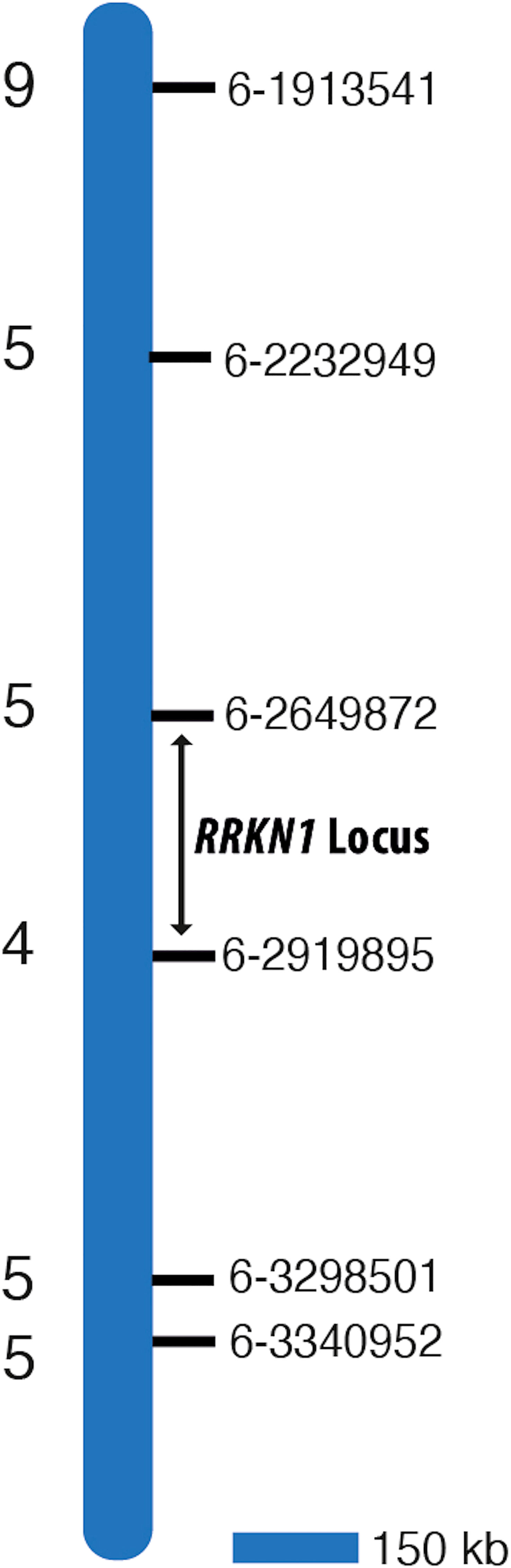
Figure 1 Map interval of RRKN1 on tomato chromosome 6. Coordinates of molecular markers used to map the RRKN1 locus on the reference tomato genome SL3 are given on the right of the bar. Numbers on the left of the bar indicate the number of recombinants in 130 F2 individuals.
The RRKN1 interval contains defense-related genes
We identified genes within the interval for RRKN1, using the ITAG annotation of tomato reference genomes SL.3 and SL.4 (Hosmani et al, 2019). There are 28 predicted genes within the RRKN1 interval according to ITAG 3.2 (Table 4) and 24 predicted genes according to ITAG version 4.0. The numbers of genes differ as genes Solyc06g008770.2 (Mi-1E, CNL4), Solyc06g008790.3 (Mi-1F, Mi-1.1) and Solyc06g008800.2 (Mi-1G, CNL6) in ITAG 3.2 disappeared in ITAG 4.0 and two genes encoding transport inhibitor response 1 protein are also missing from ITAG 3.2.
The genes Mi-1E, Mi-1F and Mi-1G within the interval fall within a clade of nematode-specific R genes, as sequence similarity searches using BLASTP against the NCBI’s Non-Redundant Proteins database revealed highest degree of similarity with R-genes previously implicated in resistance to nematodes in tomato and pepper. As tomato accession MT12 does not carry a functional allele of Mi-1.2, the most likely candidates for RRKN1 are Mi-1E, Mi-F and Mi-1G. Amino acid sequence alignments of these three putative R-proteins along with Mi-1.2 indicated these proteins are highly similar to each other but not identical (Figure 2).
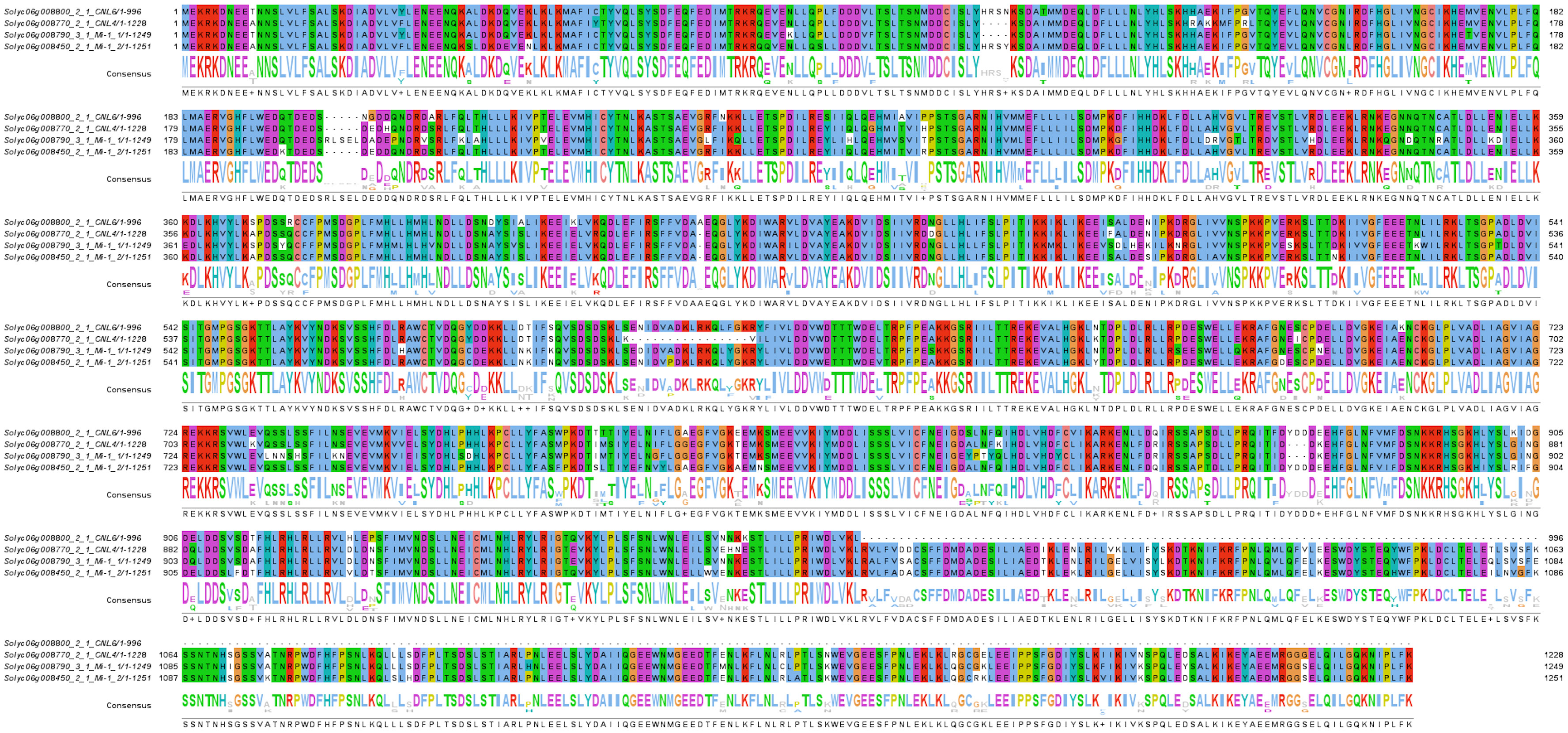
Figure 2 Multiple sequence alignment of Mi-1.2 and the three NLR proteins encoded in the 270-kb interval. Amino acid sequences were obtained from the International Tomato Genome Sequencing Project (https://solgenomics.net/organism/Solanum_lycopersicum/genome/) and aligned using Clustal Omega (Sievers et al., 2011) at the website of the European Bioinformatics Institute (McWilliam et al., 2013). The alignment image was rendered using Jalview (Waterhouse et al., 2009). The interval contains three possible candidates for RRKN1: Mi-E, Mi-F and Mi-G. In the SolGen genome annotation, these genes correspond to loci Solyc06g008770.2.1, Solyc06g008790.3.1 and Solyc06g008800.2.1 respectively. The respective GenBank protein accessions are ABI96216.1, ABI96217.1 and ABI96218.1.
RRKN1 gene region is heterozygous in parents
As the mapping population was generated by crossing two F1 parents, the genomic vicinity of the resistance gene is expected to be heterozygous. Therefore, we developed five new KASP markers were developed within the interval (Supplementary Table 2) and the markers were then used these to screen parents and the mapping population. The results confirmed the region to be heterozygous (Supplementary Table 4).
Discussion
The Mi-1.2 gene is widely used for resistance to root-knot nematodes; however, it has some significant drawbacks, including breakdown of resistance at high soil temperatures. Therefore, new resistance sources must be identified and incorporated into tomato breeding programmes. In this study, we demonstrate that resistance source MT12 does not carry a functional alle of Mi-1.2 but provides durable resistance due to RRKN1 at high soil temperatures. Additionally, MT12 does not confer resistance to naturally identified Mi-1.2-virulent isolates, indicating that RRKN1 shares a similar resistance-spectrum to Mi-1.2 but does not break down at high temperature. Also, as expected, the Seval F1 plant carrying the Mi-1.2 gene was observed to lose resistance at high soil temperatures. In previous studies, different resistance genes such as Mi-2, Mi-3, Mi-4, Mi-5, Mi-6 and Mi-9 were determined against RKNs at high soil temperatures (Cap et al., 1993; Yaghoobi et al., 1995; Veremis and Roberts, 1996a; Veremis and Roberts, 1996b; Veremis et al., 1999; Ammiraju et al., 2003).
Ammiraju et al. (2003) found the LA2157 (Mi-9) accession to be resistant to avirulent populations of M. arenaria, M. incognita and M. javanica at 25°C and 32°C. Also, resistance was provided by a single dominant gene located in the vicinity of the Mi-1.2 gene on the short arm of chromone 6. In another study, Jablonska et al. (2007) suggested that Mi-9, which is a heat-stable resistance gene, was located on chromosome 6 and may be a homologue of Mi-1. Recently, Wang et al. (2013) reported that Mi-HT, conferring resistance to M. incognita at high temperatures (32°C) in line ZN17, was a single dominant gene and located on the short arm of chromosome 6. Also, molecular data indicated that Mi-HT might be an orthologue of Mi-1 and Mi-9 or a new gene. Gene Mi-9 is located on chromosome 6 between positions 2305101 and 3327863 and Mi-HT is between 2305101 bp and 2327783 bp, based on investigating positions of published markers linked to Mi-9 and Mi-HT genes in version SL3.1 genome assembly of tomato. In the present study, we showed that the RRKN1 gene is on chromosome 6 and in a 270-kb interval between positions 2.649.872 and 2.919.895. Recently, Jiang et al. (2023) determined that the Mi-9 gene is located between nucleotides 3.113.154 and 3.116.774 on chromosome 6 of LA2157. They also showed that the similarity of Mi-1.2 and Mi-9 proteins was 97.22%.
Previously, we mapped Pvr4 in pepper (Devran et al., 2015), Frl in tomato (Devran et al., 2018) and CsCvy-1 in cucumber (Kahveci et al., 2021) using genomics coupled with the bulked segregant analysis method. In this study, to determine the location of RRKN1, we developed molecular markers to cover all chromosomes and used them to screen F2 populations. Narrowing the genomic interval depends on the number of recombinants in a given mapping population. In the mapping exercise of Frl gene in tomato, we used 542 F2 lines and mapped the gene to a 900-kb interval on chromosome 9 (Devran et al., 2018). In this study, we used only 130 F2 lines and determined a 270-kb interval for RRKN1. This improved genomic resolution may have been due to the fact that F1 lines in a commercial programme are produced from pure breeding lines, which would have undergone many recombination events due to continuous crossing to accumulate the desired traits. This in turn, may have helped identification of the desired recombinant lines for mapping. As we had a few more recombinants from either side of the flanking markers, we attempted to narrow the interval further and developed markers tightly linked to the gene. Results of implementing newly developed KASP markers proved difficult due to the heterozygous nature of the RRKN1 interval. In some cases, the number of the recombination in a region could be influenced by chromosome rearrangement and recombination suppression (Tang et al., 2008; Verlaan et al., 2011).
The interval contains three possible candidates for RRKN1: Mi-E, Mi-F and Mi-G. In the SolGen genome annotation, these genes correspond to loci Solyc06g008770.2.1, Solyc06g008790.3.1 and Solyc06g008800.2.1 respectively. The amino acid alignment of these putative disease resistance proteins show that they are very similar to each other as well as to the well-known Mi-1.2. Recent studies show that the NLR proteins work as a pair. For example, Arabidopsis RPP2 family (RPP2A, RPP2B, RPP2C and RPP2D) have been shown to be required for the recognition of ATR2 effector for the downy mildew pathogen (Kim et al., 2023). Similarly, Arabidopsis TIR-NLR RRS1 and RPS4 (Guo et al., 2020), and the rice CC-NLR pairs RGA4/RGA5 and Pik-1/Pik-2 (Zdrzałek et al., 2020) have been reported to work together. As we did not proceed to clone the functional gene for RRKN1, further studies would be required to reveal which one(s) of these three candidate genes are providing the resistance against the RKNs. Possible approaches that can be utilized may include CRISPR/Cas9 system (Erdoğan et al., 2023), virus-induced gene silencing (VIGS) method (Valentine et al., 2004), cloning each of them and transforming to a susceptible line and assaying the lines with a relevant nematode population. Once the gene is identified, a new MAS-friendly marker could be developed withing the gene to use in the breeding programme.
In summary, root-knot nematodes are an important problem for tomato growing areas. The use of resistant cultivars is one of the most attractive disease management methods. The Mi-1.2 gene has been used for a long time in tomatoes. However, Mi-1.2 gene was overcome by Mi-1.2-virulent RKN populations (Roberts, 1990; Castagnone-Sereno et al., 1994; Kaloshian et al., 1996; Devran and Söğüt, 2010) and loses its effect at high soil temperatures (Dropkin, 1969; Özalp and Devran, 2018). Molecular marker-assisted selection of a resistant gene is important for breeding programmes. In conclusion, identifying a new gene such as RRKN1 and developing molecular markers tightly linked to gene, discovered in the present study, can help generate new tomato varieties, fine-mapping and cloning of the gene. Growers can use RRKN1-carrying lines instead of tomato varieties bearing the Mi-1.2 resistance that breaks down at high soil temperature. In addition, pyramiding of RRKN1 and Mi-1.2 genes in superior tomato lines would prolong controlling of the root-knot nematodes.
Data availability statement
The datasets presented in this study can be found in online repositories. The names of the repository/repositories and accession number(s) can be found in the article/Supplementary Material.
Author contributions
ZD: Conceptualization, Data curation, Formal Analysis, Funding acquisition, Methodology, Project administration, Supervision, Validation, Writing – original draft, Writing – review & editing. TÖ: Data curation, Formal Analysis, Methodology, Writing – review & editing. DS: Formal Analysis, Writing – review & editing. MT: Conceptualization, Formal Analysis, Funding acquisition, Supervision, Writing – original draft, Writing – review & editing.
Funding
The authors declare financial support was received for the research, authorship, and/or publication of this article. This study was financially funded by The Scientific and Technological Research Council of Turkey-TUBITAK (Grant Number: 119O412).
Acknowledgments
The authors thank to The Scientific and Technological Research Council of Turkey for providing the financial support to ZD, M.Y. Genetik Tarım Tek. Lab. Tic. Ltd. Şti., providing the necessary equipments to carry out nematology testing and molecular studies, and Multi Tohum’s tomato breeders for providing the parental genotypes, F1 and F2 plants. We also thank Fatmana Doğan for her technical support in KASP analysis.
Conflict of interest
The authors declare that the research was conducted in the absence of any commercial or financial relationships that could be construed as a potential conflict of interest.
The author(s) declared that they were an editorial board member of Frontiers, at the time of submission. This had no impact on the peer review process and the final decision.
Publisher’s note
All claims expressed in this article are solely those of the authors and do not necessarily represent those of their affiliated organizations, or those of the publisher, the editors and the reviewers. Any product that may be evaluated in this article, or claim that may be made by its manufacturer, is not guaranteed or endorsed by the publisher.
Supplementary material
The Supplementary Material for this article can be found online at: https://www.frontiersin.org/articles/10.3389/fpls.2023.1267399/full#supplementary-material
References
Abd-Elgawad, M. M. M. (2022). Understanding molecular plant–nematode interactions to develop alternative approaches for nematode control. Plants 11, 2141. doi: 10.3390/plants11162141
Ammiraju, J., Veremis, J., Huang, X., Roberts, P., Kaloshian, I. (2003). The heat-stable root-knot nematode resistance gene Mi-9 from Lycopersicon Peruvianum is localized on the short arm of chromosome 6. Theor. Appl. Genet. 106, 478–484. doi: 10.1007/s00122-002-1106-y
Barragan, A. C., Weigel, D. (2021). Plant NLR diversity: the known unknowns of pan-NLRomes. Plant Cell 33, 814–831. doi: 10.1093/plcell/koaa002
Cap, G. B., Roberts, P., Thomason, I. (1993). Inheritance of heat-stable resistance to Meloidogyne incognita in Lycopersicon Peruvianum and its relationship to the Mi gene. Theor. Appl. Genet. 85, 777–783. doi: 10.1007/BF00225019
Castagnone-Sereno, P., Bongiovanni, M., Dalmasso, A. (1994). Reproduction of virulent isolates of Meloidogyne incognita on susceptible and Mi-resistant tomato. J. Nematol. 26, 324–328.
Devran, Z., Kahveci, E. (2019). Development and validation of a user-friendly KASP marker for the Sw-5 locus in tomato. Australas. Plant Pathol. 48, 503–507. doi: 10.1007/s13313-019-00651-1
Devran, Z., Kahveci, E., Hong, Y., Studholme, D. J., Tör, M. (2018). Identifying molecular markers suitable for Frl selection in tomato breeding. Theor. Appl. Genet. 131, 2099–2105. doi: 10.1007/s00122-018-3136-0
Devran, Z., Kahveci, E., Özkaynak, E., Studholme, D. J., Tör, M. (2015). Development of molecular markers tightly linked to Pvr4 gene in pepper using next-generation sequencing. Mol. Breed. 35, 1–9. doi: 10.1007/s11032-015-0294-5
Devran, Z., Sogut, M. A. (2009). Distribution and identification of root-knot nematodes from Turkey. J. Nematol. 41, 128–133.
Devran, Z., Söğüt, M. A. (2010). Occurrence of virulent root-knot nematode populations on tomatoes bearing the Mi gene in protected vegetable-growing areas of Turkey. Phytoparasitica 38, 245–251. doi: 10.1007/s12600-010-0103-y
Dropkin, V. (1969). The necrotic reaction of tomatoes and other hosts resistant to Meloidogyne: reversal by temperature. Phytopathology 59, 1632–1637.
Du, C., Jiang, J., Zhang, H., Zhao, T., Yang, H., Zhang, D., et al. (2020). Transcriptomic profiling of Solanum Peruvianum LA3858 revealed a Mi-3-mediated hypersensitive response to Meloidogyne incognita. BMC Genomics 21, 250. doi: 10.1186/s12864-020-6654-5
Erdoğan, İ., Cevher-Keskin, B., Bilir, Ö., Hong, Y., Tör, M. (2023). Recent developments in CRISPR/Cas9 genome-editing technology related to plant disease resistance and abiotic stress tolerance. Biology 12, 1037. doi: 10.3390/biology12071037
Fernandez-Pozo, N., Menda, N., Edwards, J. D., Saha, S., Tecle, I. Y., Strickler, S. R., et al. (2015). The Sol Genomics Network (SGN) from genotype to phenotype to breeding. Nucleic Acids Res. 43, 1036–1041. doi: 10.1093/nar/gku1195
Guo, H., Ahn, H. Y., Sklenar, J., Huang, J., Ma, Y., Ding, P., et al. (2020). Phosphorylation-regulated activation of the Arabidopsis RRS1-R/RPS4 immune receptor complex reveals two distinct effector recognition mechanisms. Cell Host Microbe 27, 769–781.e6. doi: 10.1016/j.chom.2020.03.008
Gururani, M. A., Venkatesh, J., Upadhyaya, C. P., Nookaraju, A., Pandey, S. K., Park, S. W. (2012). Plant disease resistance genes: current status and future directions. Physiol. Mol. Plant Pathol. 78, 51–65. doi: 10.1016/j.pmpp.2012.01.002
Hashem, M., Abo-Elyousr, K. A. (2011). Management of the root-knot nematode Meloidogyne incognita on tomato with combinations of different biocontrol organisms. Crop Protect. 30, 285–292. doi: 10.1016/j.cropro.2010.12.009
Hosmani, P. S., Flores-Gonzalez, M., van de Geest, H., Maumus, M., Bakker, L. V., Schijlen, E., et al. (2019). An improved de novo assembly and annotation of the tomato reference genome using single-molecule sequencing, Hi-C proximity ligation and optical maps. bioRxiv, 767764. doi: 10.1101/767764
Jablonska, B., Ammiraju, J. S., Bhattarai, K. K., Mantelin, S., De Ilarduya, O. M., Roberts, P. A., et al. (2007). The Mi-9 gene from Solanum arcanum conferring heat-stable resistance to root-knot nematodes is a homolog of Mi-1. Plant Physiol. 143, 1044–1054. doi: 10.1104/pp.106.089615
Jiang, L., Ling, J., Zhao, J., Yang, Y., Yang, Y., Li, Y., et al. (2023). Chromosome-scale genome assembly-assisted identification of Mi-9 gene in Solanum arcanum accession LA2157, conferring heat-stable resistance to Meloidogyne incognita. Plant Biotechnol. J. 21, 1496–1509. doi: 10.1111/pbi.14055
Kahveci, E., Devran, Z., Özkaynak, E., Hong, Y., Studholme, D. J., Tör, M. (2021). Genomic-assisted marker development suitable for Cscvy-1 selection in cucumber breeding. Front. Plant Sci. 12. doi: 10.3389/fpls.2021.691576
Kaloshian, I., Williamson, V., Miyao, G., Lawn, D., Westerdahl, B. (1996). “Resistance-breaking” nematodes identified in California tomatoes. Calif. Agric. 50, 18–19. doi: 10.3733/ca.v050n06p18
Kaloshian, I., Yaghoobi, J., Liharska, T., Hontelez, J., Hanson, D., Hogan, P., et al. (1998). Genetic and physical localization of the root-knot nematode resistance locus Mi in tomato. Mol. Gen. Genet. 257, 376–385. doi: 10.1007/s004380050660
Kim, D. S., Woods-Tör, A., Cevik, V., Furzer, O. J., Li, Y., Ma, W., et al. (2023). ATR2Cala2 from Arabidopsis-infecting downy mildew requires 4 TIR-NLR immune receptors for full recognition. bioRxiv, 538220. doi: 10.1101/2023.04.25.538220
Li, H., Handsaker, B., Wysoker, A., Fennell, T., Ruan, J., Homer, N., et al. (2009). The sequence alignment/map format and SAMtools. Bioinformatics 25, 2078–2079. doi: 10.1093/bioinformatics/btp352
Marquez, J., Hajihassani, A. (2023). Successional effects of cover cropping anddeep tillage on suppression of plant-parasitic nematodes and soilborne fungal pathogen. Pest Manage. Sci. 79, 2737–2747. doi: 10.1002/ps.7450
Mchale, L., Tan, X., Koehl, P., Michelmore, R. W. (2006). Plant NBS-LRR proteins: adaptable guards. Genome Biol. 7, 212. doi: 10.1186/gb-2006-7-4-212
McWilliam, H., Li, W., Uludag, M., Squizzato, S., Park, Y. M., Buso, N., et al. (2013). Analysis tool web services from the EMBL-EBI. Nucleic Acids Res. 41 (Web Server issue), W597–W600. doi: 10.1093/nar/gkt376
Milligan, S. B., Bodeau, J., Yaghoobi, J., Kaloshian, I., Zabel, P., Williamson, V. M. (1998). The root knot nematode resistance gene Mi from tomato is a member of the leucine zipper, nucleotide binding, leucine-rich repeat family of plant genes. Plant Cell 10, 1307–1319. doi: 10.1105/tpc.10.8.1307
Nas, Y., Özalp, T., Devran, Z. (2023). Screening of Urfa pepper landraces for resistance to Meloidogyne incognita. J. Plant Dis. Prot. 130, 77–83. doi: 10.1007/s41348-022-00673-w
Öçal, S., Özalp, T., Devran, Z. (2018). Reaction of wild eggplant Solanum torvum to different species of root-knot nematodes from Turkey. J. Plant Dis. Prot. 125, 577–580. doi: 10.1007/s41348-018-0167-3
Özalp, T., Devran, Z. (2018). Response of tomato plants carrying Mi-1 gene to Meloidogyne incognita (Kofoid & White 1919) chitwood 1949 under high soil temperatures. Turkish J. Entomol. 42, 313–322. doi: 10.16970/entoted.467189
Phani, V., Khan, M. R., Dutta, T. K. (2021). Plant-parasitic nematodes as a potential threat to protected agriculture: Current status and management options. Crop Prot. 144, 105573. doi: 10.1016/j.cropro.2021.105573
Rawal, S. (2020). A review on root-knot nematode infestation and its management practices through different approaches in tomato. Trop. Agroecosystem 1, 92–96. doi: 10.26480/taec.02.2020.92.96
Roberts, P. (1990). “Resistance to nematodes: definitions, concepts, and consequences,” in Methods for evaluating plant species for resistance to plant-parasitic nematodes. Ed. Star, J. L. (Maryland, USA: Society of Nematologists, Hyattsville), 1–15.
Roberts, P., Thomason, J. (1986). Variability in reproduction of isolates of Meloidogyne incognita and M. javanica on resistant tomato genotypes. Plant Dis. 70, 547–551. doi: 10.1094/PD-70-547
Robinson, J. T., Thorvaldsdóttir, H., Winckler, W., Guttman, M., Lander, E. S., Getz, G., et al. (2011). Integrative genomics viewer. Nat. Biotechnol. 29, 24–26. doi: 10.1038/nbt.1754
Seah, S., Williamson, V. M., Garcia, B. E., Mejia, L., Salus, M. S., Martin, C. T., et al. (2007). Evaluation of a codominant SCAR marker for detection of the Mi-1 locus for resistance to root-knot nematode in tomato germplasm. Tomato Genet. Cooperative Rep. 57, 37–40.
Sievers, F., Wilm, A., Dineen, D. G., Gibson, T. J., Karplus, K., Li, W., et al. (2011). Fast, scalable generation of high-quality protein multiple sequence alignments using Clustal Omega. Mol. Syst. Biol. 7, 539. doi: 10.1038/msb.2011.75
Talavera, M., Verdejo-Lucas, S., Ornat, C., Torres, J., Sorribas, F. (2009). Crop rotations with Mi gene resistant and susceptible tomato cultivars for management of root-knot nematodes in plastic houses. Crop Prot. 28, 662–667. doi: 10.1016/j.cropro.2009.03.015
Tang, X., Szinay, D., Lang, C., Ramanna, M. S., van der Vossen, E. A., Datema, E., et al. (2008). Cross-species bacterial artificial chromosome-fluorescence in situ hybridization painting of the tomato and potato chromosome 6 reveals undescribed chromosomal rearrangements. Genetics 180, 1319–1328. doi: 10.1534/genetics.108.093211
Valentine, T., Shaw, J., Blok, V. C., Phillips, M. S., Oparka, K. J., Lacomme, C. (2004). Efficient virus-induced gene silencing in roots using a modified tobacco rattle virus vector. Plant Physiol. 136, 3999–4009. doi: 10.1104/pp.104.051466
Veremis, J., Roberts, P. (1996a). Identification of resistance to Meloidogyne javanica in the Lycopersicon Peruvianum complex. Theor. Appl. Genet. 93, 894–901. doi: 10.1007/BF00224091
Veremis, J., Roberts, P. (1996b). Relationships between Meloidogyne incognita resistance genes in Lycopersicon Peruvianum differentiated by heat sensitivity and nematode virulence. Theor. Appl. Genet. 93, 950–959. doi: 10.1007/BF00224098
Veremis, J., Roberts, P. (2000). Diversity of heat-stable genotype specific resistance to Meloidogyne in Maranon races of Lycopersicon Peruvianum complex. Euphytica 111, 9–16. doi: 10.1023/A:1003776201585
Veremis, J., Van Heusden, A., Roberts, P. (1999). Mapping a novel heat-stable resistance to Meloidogyne in Lycopersicon Peruvianum. Theor. Appl. Genet. 98, 274–280. doi: 10.1007/s001220051068
Verlaan, M. G., Szinay, D., Hutton, S. F., de Jong, H., Kormelink, R., Visser, R. G., et al. (2011). Chromosomal rearrangements between tomato and Solanum Chilense hamper mapping and breeding of the TYLCV resistance gene Ty-1. Plant J. 68, 1093–1103. doi: 10.1111/j.1365-313X.2011.04762.x
Wang, Y., Yang, W., Zhang, W., Han, Q., Feng, M., Shen, H. (2013). Mapping of a heat-stable gene for resistance to southern root-knot nematode in Solanum lycopersicum. Plant Mol. Biol. Rep. 31, 352–362. doi: 10.1007/s11105-012-0505-8
Waterhouse, A. M., Procter, J. B., Martin, D. M. A., Clamp, M., Barton, G. J. (2009). Jalview Version 2 - A multiple sequence alignment editor and analysis workbench. Bioinformatics 25, 1189–1191. doi: 10.1093/bioinformatics/btp033
Williamson, V. M., Hussey, R. S. (1996). Nematode pathogenesis and resistance in plants. Plant Cell 8, 1735–1745. doi: 10.1105/tpc.8.10.1735
Wu, W. W., Shen, H. L., Yang, W. C. (2009). Sources for heat-stable resistance to southern root-knot nematode (Meloidogyne incognita) in Solanum lycopersicum. Agric. Sci. China 8, 697–702. doi: 10.1016/S1671-2927(08)60267-9
Yaghoobi, J., Kaloshian, I., Wen, Y., Williamson, V. (1995). Mapping a new nematode resistance locus in Lycopersicon Peruvianum. Theor. Appl. Genet. 91, 457–464. doi: 10.1007/BF00222973
Keywords: high soil temperature, gene mapping, Meloidogyne incognita, RRKN1, SNP
Citation: Devran Z, Özalp T, Studholme DJ and Tör M (2023) Mapping of the gene in tomato conferring resistance to root-knot nematodes at high soil temperature. Front. Plant Sci. 14:1267399. doi: 10.3389/fpls.2023.1267399
Received: 26 July 2023; Accepted: 21 September 2023;
Published: 10 October 2023.
Edited by:
Shiming Liu, Chinese Academy of Agricultural Sciences, ChinaReviewed by:
Juan Emilio Palomares-Rius, Spanish National Research Council (CSIC), SpainYingpeng Han, Northeast Agricultural University, China
Copyright © 2023 Devran, Özalp, Studholme and Tör. This is an open-access article distributed under the terms of the Creative Commons Attribution License (CC BY). The use, distribution or reproduction in other forums is permitted, provided the original author(s) and the copyright owner(s) are credited and that the original publication in this journal is cited, in accordance with accepted academic practice. No use, distribution or reproduction is permitted which does not comply with these terms.
*Correspondence: Zübeyir Devran, emRldnJhbkBha2Rlbml6LmVkdS50cg==; Mahmut Tör, bS50b3JAd29yYy5hYy51aw==
†ORCID: Zübeyir Devran, orcid.org/0000-0001-7150-284X
Tevfik Özalp, orcid.org/0000-0003-1620-9020
David J. Studholme, orcid.org/0000-0002-3010-6637
Mahmut Tör, orcid.org/0000-0002-4416-5048