- Chromosome Research Institute, Department of Chemistry & Life Science, Sahmyook University, Seoul, Republic of Korea
Introduction: Tandem repeats (TRs) occur abundantly in plant genomes. They play essential roles that affect genome organization and evolution by inducing or generating chromosomal rearrangements such as duplications, deletions, inversions, and translocations. These impact gene expression and chromosome structure and even contribute to the emergence of new species.
Method: We investigated the effects of TRs on speciation in Senna genus by performing a comparative analysis using fluorescence in situ hybridization (FISH) with S. tora-specific TR probes. We examined the chromosomal distribution of these TRs and compared the genome sizes of seven Senna species (estimated using flow cytometry) to better understand their evolutionary relationships.
Results: Two (StoTR03_159 and StoTR04_55) of the nine studied TRs were not detected in any of the seven Senna species, whereas the remaining seven were found in all or some species with patterns that were similar to or contrasted with those of S. tora. Of these studies species, only S. angulata showed significant genome rearrangements and dysploid karyotypes resembling those of S. tora. The genome sizes varied among these species and did not positively correlate with chromosome number. Notably, S. angulata had the fewest chromosomes (2n = 22) but a relatively large genome size.
Discussion: These findings reveal the dynamics of TRs and provide a cytogenetic depiction of chromosomal rearrangements during speciation in Senna. To further elucidate the dynamics of repeat sequences in Senna, future studies must include related species and extensive repeatomic studies, including those on transposable elements.
1 Introduction
DNA tandem repeats (TRs) are abundant in heterochromatic regions within plant genomes, such as the (peri)centromere and (sub)telomere. These regions are hotspots of chromosomal rearrangement during genomic perturbations and may lead to the development of new species with altered chromosomal numbers or organization (Schubert and Lysak, 2011; Garrido-Ramos, 2017; Pellestor and Gatinois, 2018; Rosato et al., 2018; Hartley and O’neill, 2019). TRs and chromosomal rearrangements show close association because TRs both cause and result from chromosomal rearrangements and contribute to chromosomal evolution and speciation (Sousa and Renner, 2015; Louzada et al., 2020). The abundance and distribution of TRs vary among species, making them useful cytotaxonomic markers of phylogenetic relationships (Perumal et al., 2017). Additionally, chromosomal fragmentation or fusion may result in different basic chromosome numbers within a genus, which can result in dysploidy that could be ascending (species with more chromosomes) or descending (species with fewer chromosomes) (Mandáková et al., 2010; Schubert and Lysak, 2011; Mandáková et al., 2019). Tracking the distribution of these repeats across chromosomes and analyzing the dynamics of different repeat families may provide insights into the genomic history of closely related taxa (Perumal et al., 2017; Louzada et al., 2020).
The genus Senna Mill. (formerly Cassia L.) belongs to family Fabaceae and comprises approximately 350 species of herbs, shrubs, and trees that are extensively dispersed in tropical and subtropical regions (Azani et al., 2017; Bradley Morris et al., 2019). Senna species have been used for various economic and medicinal applications such as treating diabetes, microbial infections, skin diseases, gastrointestinal disorders, and inflammation (Oliur Rahman et al., 2013; Ongchai et al., 2019; Kang et al., 2020b). They contain a diverse range of important metabolites (alkaloids, anthraquinones, flavonoids, tannins, glycosides, steroids, terpenoids, and saponins) and display a wide range of in vitro and in vivo pharmacological activities (antidiabetic, anti-gonorrhea, antimicrobial, antioxidant, antipyretic, antinociceptive, antidepressant, and anti-inflammatory effects) (Puri, 2018; Kang et al., 2020a; Oladeji et al., 2021). However, the genus Senna presents challenges for cytogenetic studies owing to the diverse morphological and ecological characteristics of its members (Cordeiro and Felix, 2017; Nguyen et al., 2021; Ta et al., 2021; Waminal et al., 2021). Furthermore, Senna exhibits high levels of chromosomal variability with reported chromosome numbers ranging from 14–120 (Cordeiro and Felix, 2017). This variability complicates efforts to identify consistent patterns or relationships among Senna species and hampers the development of reliable cytogenetic markers for taxonomic and evolutionary studies.
Fluorescence in situ hybridization (FISH) is a powerful tool used in plant cytogenetic studies. It aids in the visualization of the DNA probes located on chromosomes within the nucleus, revealing information pertaining to chromosome structure, genome organization, and gene expression (Iovene et al., 2008; Waminal et al., 2018). FISH is particularly useful in plant breeding programs for investigating the evolutionary relationships between plant species and studying the impact of environmental stressors on chromosome structure and behavior (Sassi et al., 2019; Falistocco, 2020; de Moraes et al., 2021; Waminal et al., 2021).
Estimating genome size is essential for taxonomic purposes such as clarifying taxonomy and nomenclature, evaluating interspecific hybrid seedlings, and identifying ploidy levels (Bennett and Leitch, 2005). As genome size can be a distinguishing feature of individual species, genome size analysis assists in recognizing whole-genome duplication (WGD) and other genomic evolution events (Doležel et al., 2007; Bourge et al., 2018). Flow cytometry is now the preferred technique for genome size measurement (Bourge et al., 2018). Compared with alternative techniques such as Feulgen densitometry or genome sequencing, flow cytometry offers advantages such as simplified sample preparation, high throughput, and ability to estimate genome size, nuclear replication state, ploidy, and endopolyploidy levels (Doležel et al., 2007).
Exploring the chromosomal distribution of TRs via the FISH technique and comparative genomic analysis across multiple Senna species is pivotal for addressing cytogenetic research challenges within this genus. Notably, Senna tora L. (Roxb) (syn. Cassia tora L.) emerges as a widespread and representative species within the genus, subject to comprehensive genome sequencing to enhance our understanding of its biological evolution and relationship with other Senna species (Puri, 2018; Kang et al., 2020a; Waminal et al., 2021). Moreover, TRs are discerned to actively mediate the significantly rearranged descending dysploid karyotype of S. tora. Consequently, this study engaged in a comparative analysis, employing FISH alongside S. tora -specific pre-labeled oligo probes (PLOPs) and compared the genome sizes of several related Senna species. These selected species were S. angulata (Vogel) H. S. Irwin & Barneby, S. artemisioides subsp. petiolaris (DC.) Randell, S. artemisioides nothosubsp. sturtii (R.Br.) Randell, S. hirsuta var. hirta (Benth.) H. S. Irwin & Barneby, S. lindheimeriana (Scheele), S. pallida (Vahl) H. S. Irwin & Barneby, and S. sophera (L.) Roxb. which included diploid, dysploid, and polyploid karyotypes, provide a better understanding of whether the repeats identified are specific to S. tora or are conserved within the genus and scrutinize the impact of TRs on the speciation process within the Senna genus. Our ambition was to amass data and potentially yield cytogenetic evidence for historical, extensive chromosomal rearrangements and to enable a deeper comprehension of the evolutionary kinships among the species.
2 Materials and methods
2.1 Plant materials
Germinated seeds belonging to seven Senna species (mentioned in Tables 1, 2) were provided by the National Plant Germplasm System (USDA, USA) and Rare Palm Seeds (Germany). The root tips were collected and treated with two mM 8-hydroxyquinoline at 18°C for 5 h to arrest cell growth at metaphase. The roots were fixed in Carnoy’s solution and stored in 70% ethanol until needed for chromosomal preparation.
2.2 Chromosome spread preparation
Sporophytic metaphase chromosome slides was prepared based on the method described by Waminal and Kim (2012) with some modifications. Briefly, small pieces of the meristematic tips (approximately 2 mm) were treated with a pectolytic enzyme solution containing 2% Cellulase RS (Phytotechnology Laboratories, Lenexa, KS, USA) and 1% Pectolyase Y-23 (Duchefa, Haarlem, The Netherlands) in a 100 mM citrate buffer for 2 h at 37°C. After washing with distilled water, the roots were placed in chilled Carnoy’s solution and vortexed for 30 s at room temperature. The pellet obtained was suspended in an acetoethanol mixture, and the cellular suspension was pipetted onto glass slides that had been prewarmed in a humid chamber. The slides were air-dried, fixed in 2% formaldehyde (Merck Schuchardt OHG, Hohenbrunn, Germany) for 5 min, dipped in distilled water, and dehydrated using increasing concentrations of ethanol (70%, 90%, and 100%).
2.3 Repeat mining, probes preparation, and fluorescence in situ hybridization
The paired-end reads of the genome of S. tora were obtained and used for quality trimming, read sampling, and repeat clustering. S. tora-specific TRs were identified in our previous study (Waminal et al., 2021) using low-coverage sequences and short-read clustering with TAREAN (Novák et al., 2017). All the prelabeled oligonucleotide probes (PLOPs) used for FISH analysis in this study are listed in Table 3.
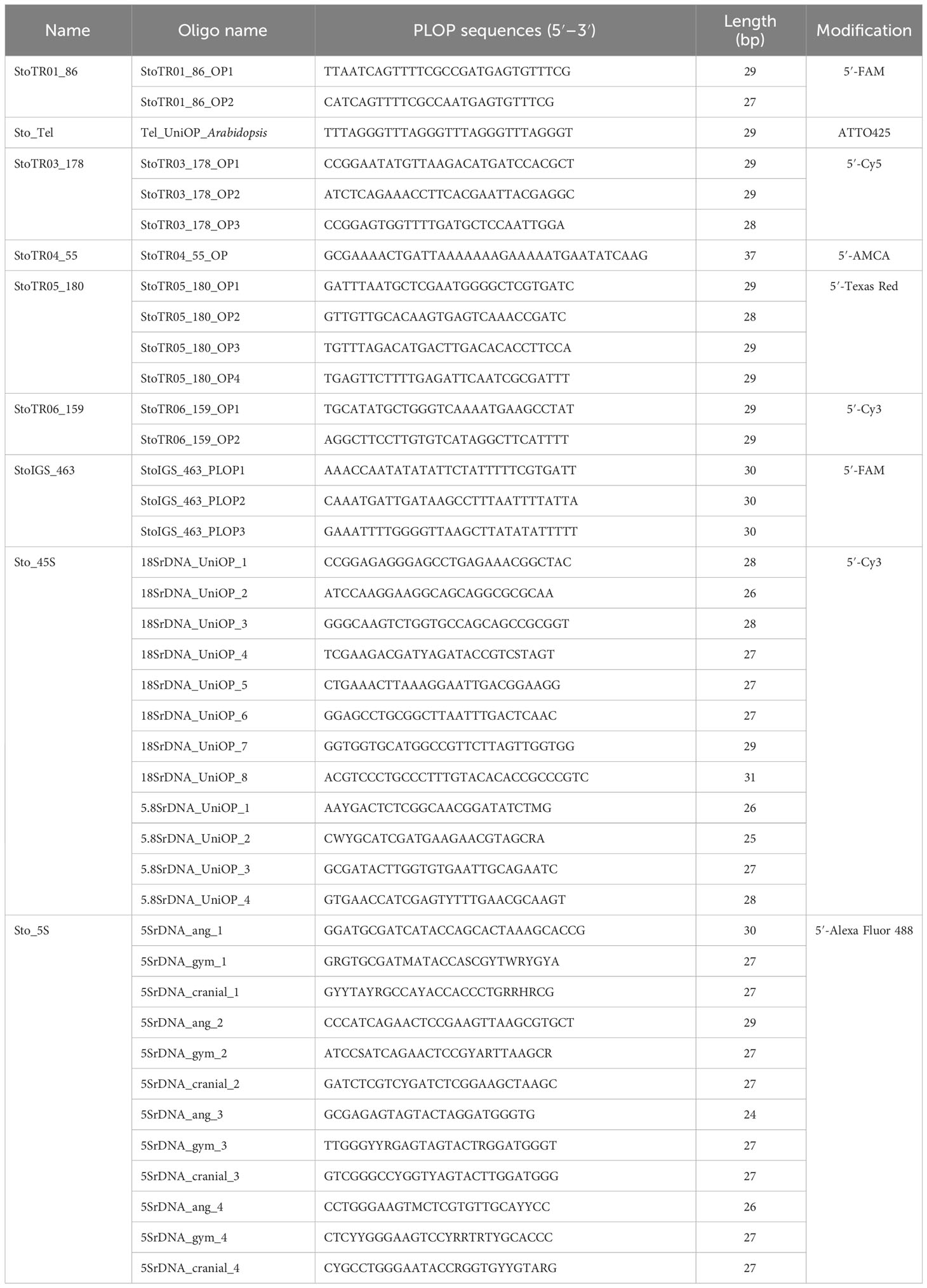
Table 3 List of pre-labeled oligonucleotide probes (PLOPs) used in this study (Waminal et al., 2021).
A 40 µL hybridization mixture was prepared by adding 100% formamide, 50% dextran sulfate, 20× SSC, 50 ng/µL of each probe, and Sigma water, followed by denaturation at 80°C and incubation at 37°C overnight. This hybridization mixture was used to perform FISH. The slides prepared earlier were washed with 2× SSC at 22-25°C (RT) and dehydrated consecutively in 70%, 90%, and 100% ethanol for 3 min each time. Finally, they were counterstained with DAPI-Vectashield (Vector Laboratories, Newark, CA, USA) and visualized with an Olympus BX53 (Olympus, Tokyo, Japan) fluorescence microscope system equipped with a Leica DFC365 FS CCD camera (Leica Microsystems, Wetzlar, Germany). The images were captured using the Cytovision software (Leica Microsystems) and enhanced using Adobe Photoshop CS6 (Adobe Inc., San Jose, California, USA). The chromosomes were analyzed using ImageJ 1.2 software and paired based on FISH signals, chromosome length, centromere position and morphological characteristics. Chromosome typing was performed as described previously by Levan et al. (1964).
2.4 Genome size measurement and assessment of nuclear DNA content using flow cytometry
The total nuclear DNA content (2C-DNA value) of the seven Senna species were measured using flow cytometry by following the method outlined by Bourge et al. (2018). Briefly, fresh leaves were collected from ten individuals of each species and mixed with an internal reference standard (Dendropanax morbifera, 2C DNA = 4.09 pg). In a petri dish, the samples were treated with the isolation LB01 buffer and passed through two nylon mesh filters (CellTrics Filters, Sysmex Asia Pacific, Singapore) with pore sizes of 50 µm and 20 µm, respectively. The filtered nuclei were stained with propidium iodide (Sigma-Aldrich, St. Louis, MO, USA, cat. no. P4170; Molecular Probes; cat. no. P3566) and RNase A (Sigma-Aldrich, St. Louis, MO, USA, cat. no. R5000) and analyzed using a CytoFLEX flow cytometer (Beckman Coulter, California, USA). The DNA content of the samples was computed by analyzing the peaks of the standards and samples using CytoExpert v2.3 software (Beckman Coulter Inc., Pasadena, CA, USA). The 2C values were calculated by estimating the linear fluorescence intensity of the stained nuclei for each species and the internal standard. The relative genome size was determined using a formula following Nguyen et al. (2023). The coefficient of variation (CV) was below 3% on average and did not exceed 7%.
3 Results
3.1 Chromosome counts
Although 2n = 28 has been the predominantly reported number of chromosomes in most Senna species, variations in chromosome number occur due to polyploidy or disploidy, resulting in 2n = 56 (S. artemisiodes subsp. petiolaris and S. artemisioides nothosubsp. sturtii) or 2n = 22 (S. angulata) chromosomes, respectively. In this study, S. angulata exhibited a diploid chromosome count of 2n = 22, contrasting with prior findings of 2n = 26 reported by Biondo et al. (2005). Notably, this is not the inaugural documentation of chromosome numbers for S. artemisiodes or S. hirsuta. However, our research marks the maiden report concerning chromosome numbers for distinct variants of these species, encompassing S. artemisiodes subsp. petiolaris and S. artemisioides nothosubsp. sturtii (2n = 56), as well as S. hirsuta var. hirta (2n = 28). Meanwhile, S. lindheimeriana, S. pallida and S. sophera displayed a consistent chromosome count of 28, aligning with previously published data.
3.2 Distribution of nine S. tora-TR probes
FISH analysis of the nine TRs examined showed that two were not present in any of the seven Senna species (StoTR06_159 and StoTR04_55), whereas the other seven were detected in all or some of the species (Table 1). The 5S rDNA, 45S rDNA, and telomeric repeats were present in all species; StoTR01_86 was present in all species except S. pallida; and StoTR01_178 and StoTR06_180 were present in only two and one species, respectively. Furthermore, StoIGS_463 was present only in S. sophera (Figures 1, 2; Table 1).
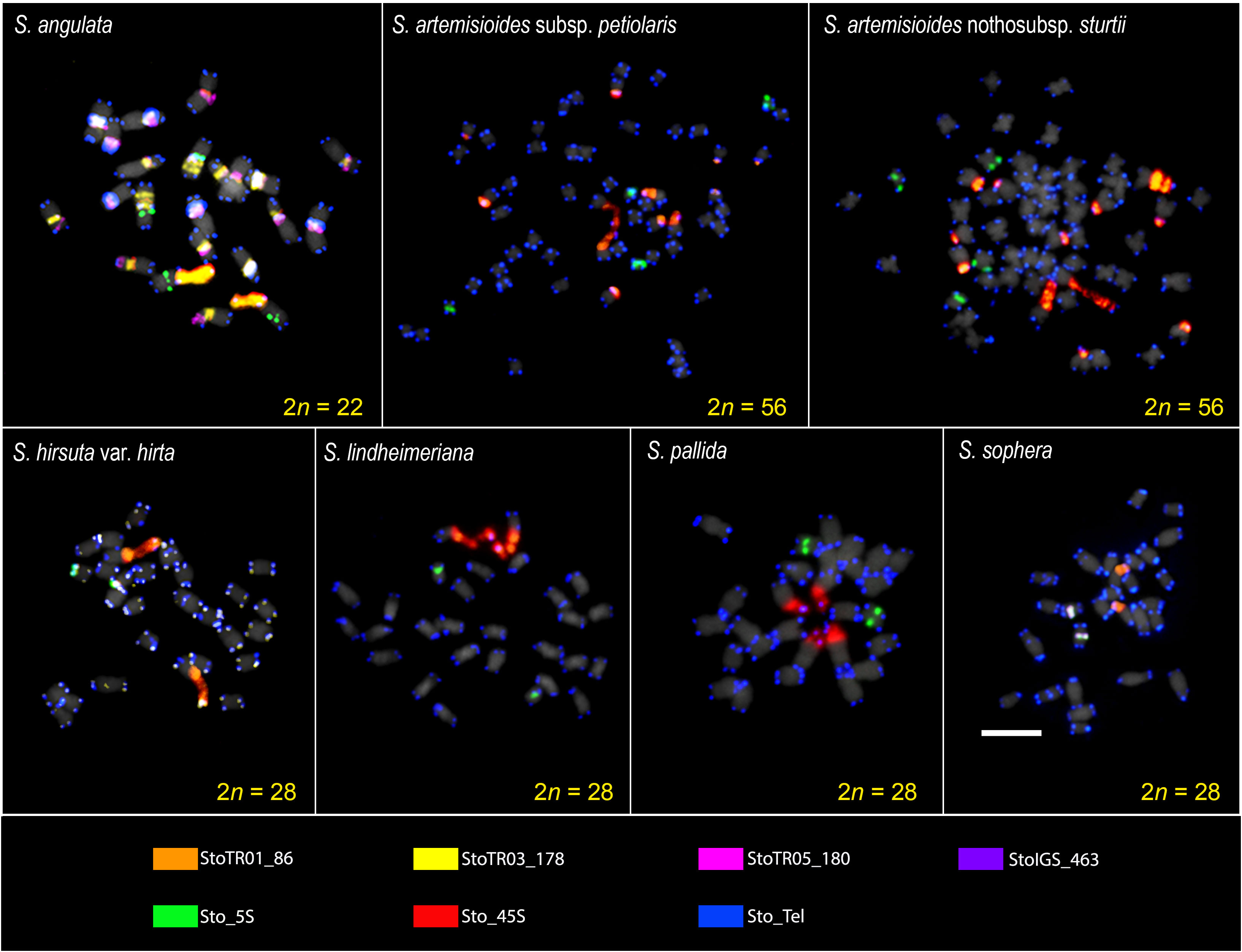
Figure 1 FISH images of S. tora-TRs on metaphase chromosomes of the seven Senna species. Seven TRs showed signals in all or some species with varied patterns. The signal patterns of individual probes are shown in Supplementary Data (Figures 1S–5S). Scale bar = 5 µm.
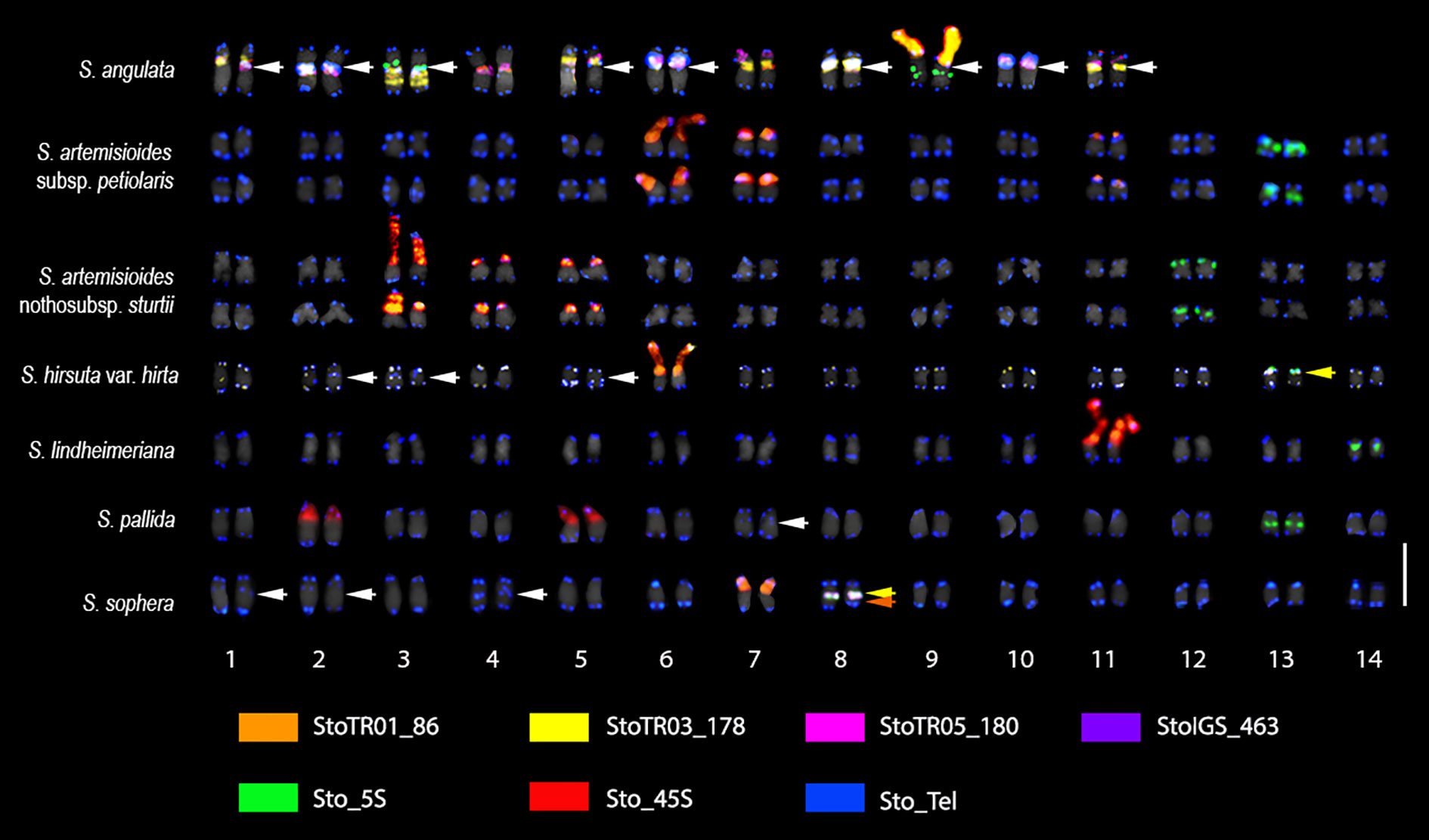
Figure 2 FISH karyogram of seven Senna species obtained using S. tora-TRs. StoTR01_86 is mainly colocalized at 45S rDNA sites in all species except in S. pallida, which did not carry the signal; S. hirsuta and S. sophera show an additional signal pair colocalized at the 5S rDNA region (yellow arrows). White arrows indicate ITRs in S. angulata, S. hirsuta, S. pallida, and S. sophera. The orange arrow shows StoIGS_463 signals colocalized with 5S rDNA in S. sophera. StoTR05_180 is present only in S. angulata at subcentromeric sites of nine chromosomes. StoTR03_178 is shown at the centromeric regions of S. angulata chromosomes; in contrast, they are observed at the subtelomeric sites in S. hirsuta. The karyogram of each species showing the individual TR distribution are shown in Supplementary Data (Figures 6S–12S). Scale bar = 5 µm.
When examining seven different Senna species, the 45S rDNA loci exhibited higher diversity than the 5S rDNA. Except for S. angulata, all species were found to have a single pair of Sto_5S rDNA in the pericentromeric or interstitial regions of the short arms of their respective chromosomes. However, in the case of S. hirsuta, Sto_5S rDNA subtelomeric signals were observed. Sto_45S rDNA was detected in one, two, or three distinct loci in these species. S. angulata was unique in exhibiting two pairs of 5S rDNA, while all its chromosomes displayed 45S rDNA signals in the (peri)centromeric regions, in addition to one pair of NOR signals. All seven species demonstrated Sto_Tel signals at the terminal regions of all chromosomes. Beyond these canonical sites, interstitial telomeric repeat (ITR) signals were identified in S. angulata, S. hirsuta, S. pallida, and S. sophera. Specifically, S. angulata displayed ITR signals in the (sub)centromeric regions of nine chromosomes. Conversely, ITRs were observed in short arms of chromosomes 2 and 3 and long arm of chromosome 5 in S. hirsuta, the short arm of chromosome 7 in S. pallida, and the short arms of chromosomes 1, 2, and 4 in S. sophera.
The distribution of StoTR01_86 in the chromosomes of the seven Senna species was examined in the present study and revealed to be completely different from that observed in S. tora, which harbors StoTR01_86 in the pericentromeric regions of all chromosomes. However, for six species of Senna studied here, it was found colocalized with all 45S rDNA loci, and S. pallida did not show any signal for StoTR01_86. Notably, S. hirsuta and S. sophera displayed an extra pair of StoTR01_86, which was colocalized at the site of the 5S rDNA signals. These findings are illustrated in Figures 1, 2 and summarized in Table 1.
In S. tora, both StoTR03_178 and StoTR05_180 exhibited a centromeric distribution across all chromosomes. However, in S. angulata, StoTR03_178 signals were observed at the centromeres of all chromosomes except for two, one of which carried the 45S rDNA signal. In this species, StoTR03_178 was also detected at pericentromeric regions in all chromosomes except the one with the 45S rDNA signal, where it was found at the NOR sites. In contrast, S. hirsuta displayed StoTR05_180 signals that colocalize with Sto_tel at chromosome termini and some interstitial regions.
Finally, in S. sophera, we identified only one pair of StoIGS_463 signals colocalized with the 5S rDNA signal, whereas in S. tora, these signals were exclusively located at the NOR site.
3.3 Flow cytometric genome size estimation
Flow cytometric analysis of the genome size (2C-value) of all seven Senna species using Dendropanax morbifera (2C = 4.09 pg) as an internal standard produced histograms with well-defined peaks and low coefficients of variation (< 3.0%) (Figure 3). This supported the reliability of flow cytometric assessments. The genome size varied among the Senna species with values ranging from 2C = 1.29 pg (631.27 Mb) in S. hirsuta to 2C = 2.43 pg (1190.07 Mb) in S. artemisioides nothosubsp. sturtii. In fact, the DNA content varied even among the species with the same number of chromosomes, that is, 2n = 28 (S. lindheimeriana, S. hirsuta, S. pallida, and S. sophera) and 2n = 56 (S. artemisioides subsp. petiolaris and S. artemisioides nothosubsp. sturtii). Notably, S. angulata, which had the fewest chromosomes (2n = 22), has a relatively large genome size, ranking it second among the seven species ahead of S. artemisioides subsp. petiolaris (2n = 56) (Figure 1; Table 2).
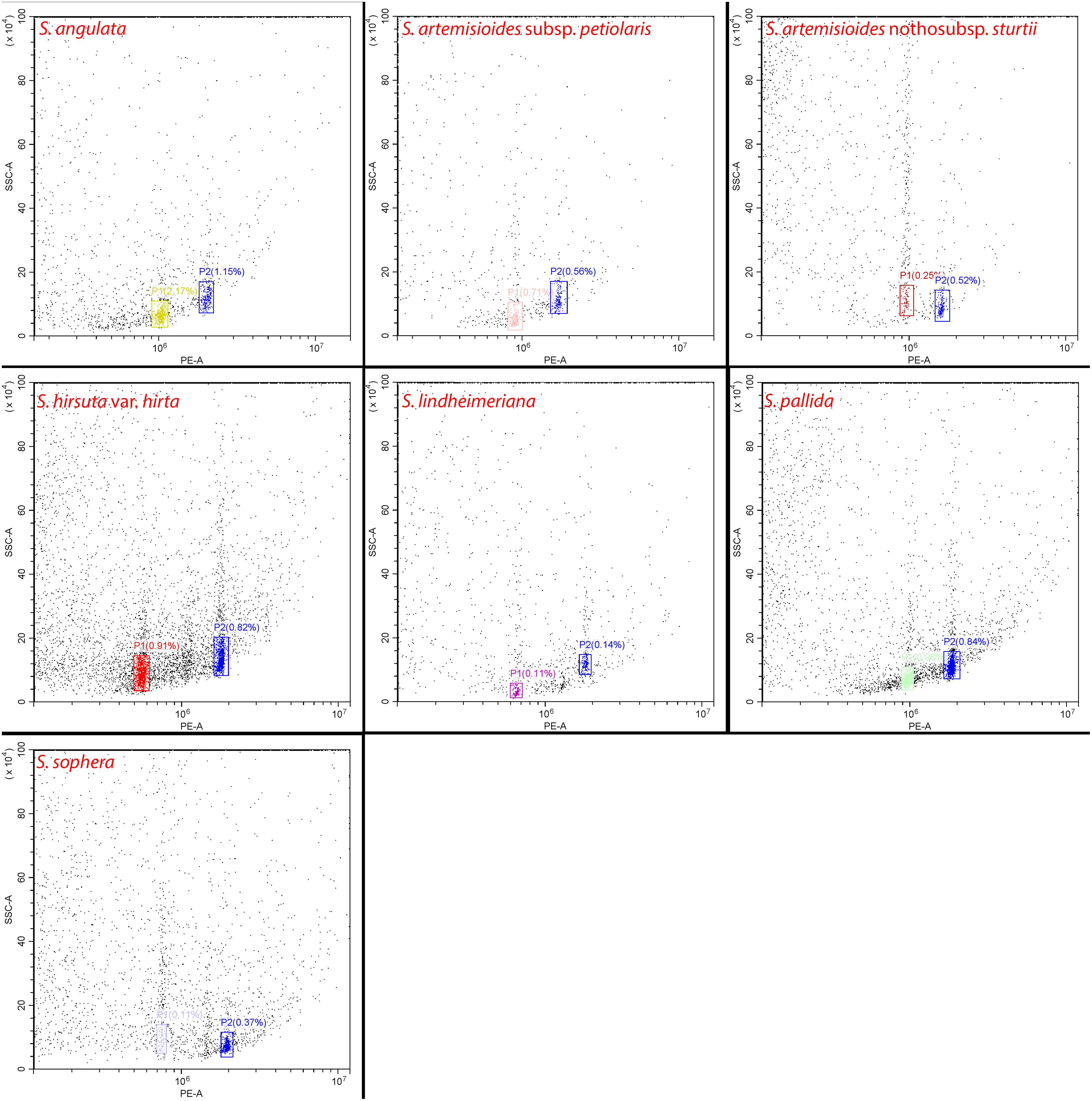
Figure 3 Flow cytometric dot plots of relative fluorescence intensities of propidium iodide-stained nuclei isolated from seven Senna species and internal standard Dendropanax morbifera (in blue, 2C = 4.09 pg).
4 Discussion
Karyotypic data play a crucial role in assessing genetic relationships, shedding light on the origin and divergence of species (Bhat and Wani, 2017). This information encompasses critical factors such as chromosome number, size, morphology, satellite DNA position, and chromosome banding patterns (Guerra, 2008). When comparing the findings to previous reports (Irwin and Turner, 1960; Biondo et al., 2005), it becomes evident that S. angulata exhibits varying chromosome counts. This discrepancy could stem from possible botanical misidentification, variations in sample accession numbers across different studies, or inherent genetic diversity within the species (Guerra, 2008). Senna species have traditionally been documented with a basic chromosome number of x = 14 being the most common (Chaulagain and Sakya, 2002; Resende et al., 2014), often considered a polyploid derivative of x = 7, a characteristic shared across all Caesalpinioideae (Elaine et al., 2005). Our analysis identified a chromosome number of 2n = 28 in four of the seven Senna species examined. However, S. angulata showed a chromosome number of 2n = 22. This discrepancy may be attributed to dysploidization events, such as chromosome fusion, occurring during Senna evolution (Elaine et al., 2005; Ferreira et al., 2010), also observed in S. tora (Waminal et al., 2021). It’s important to note that interspecies chromosome variation within a genus is not unique to Senna and has been observed in other genera like Brassica, Cucumis, Nothoscordum, and Brachyscome (Maluszynska and Heslop-Harrison 1993; Watanabe et al., 1995; Koo et al., 2010). Dysploidization, as seen in this case, is a recognized driver for speciation (Freyman and Höhna, 2018). Furthermore, our study unveiled a chromosome number of 2n = 56 in S. artemisioides subsp. petiolaris and S. artemisioides nothosubsp. sturtii. Such an observation suggests that polyploidy events may play a role in the speciation of these particular Senna subspecies.
The 45S rDNA repeats show complex distribution and organization patterns in plant genomes with wide variation between species (Roa and Guerra, 2012). Previous studies have investigated this phenomenon by analyzing the localization patterns on chromosomes in several plant species such as Arabidopsis thaliana, Brassica rapa, Cucumis species, Lilium distichum, and Solanum chacoense (Fukui et al., 1998; Hwang et al., 2015; Zhang et al., 2016). The mechanisms underlying rDNA distribution and homogenization are diverse and include transposition, unequal crossing-over, and gene conversion. In the present study, S. angulata presented 45S rDNA signals in both the NOR and (peri)centromeric regions of the chromosomes, suggesting that multiple clusters of rDNA repeats were scattered throughout the genome. As a tandemly repeating locus, rDNA is vulnerable to copy-number mutations, especially when exposed to topological stress resulting from the unwinding of the helical DNA structure and collisions between replication and transcription machinery due to frequent transcription activities (Salim and Gerton, 2019). Laboratory studies have consistently shown that exposure to various stressors can induce rapid changes in rDNA copy numbers (Kobayashi, 2011; Paredes et al., 2011; Aldrich and Maggert, 2015; Jack et al., 2015; Salim et al., 2017), highlighting the environmental sensitivity of the rDNA locus (Salim and Gerton, 2019). Furthermore, rDNA content is intimately connected to genome stability and susceptibility to stress (Kobayashi and Sasaki, 2017). This relationship may elucidate the expansion of 45S rDNA copies in all chromosomes of S. angulata, which is a dysploid karyotype that may have experienced numerous rearrangements during speciation, adaptation, survival, and evolution. The homogenization of these repeats may have occurred over time through mechanisms similar to those observed in other plant species, like Atropa belladonna, Thinopyrum intermedium (Mahelka et al., 2013; Volkov et al., 2017). In fact, transposition, unequal crossover events, and homogenization through gene conversion are possible mechanisms that may explain the observed localization and distribution of rDNA repeats in S. angulata. The presence of NOR signals on multiple chromosomes in S. artemisioides subsp. petiolaris and S. artemisioides notosubsp. sturtii suggest that they may have undergone chromosomal rearrangements, resulting in the duplication of the NOR regions. Further research is required to determine the mechanisms underlying the localization and distribution of rDNA repeats in these plant species.
The presence of StoTR01_86 at the 45S rDNA locus in most Senna species studied, except S. pallida, suggests its involvement in chromosomal rearrangements following the divergence of Senna species from their common ancestor, with the karyotype represented by S. pallida. This process may have led to the correction of error StoTR01_86 which was also observed in S. siamea in the study by Ta et al. (2021) (Table 1). Subsequently, specific chromosomal rearrangements in S. tora may have caused the recent movement of StoTR01_86 from the IGS region to the pericentric region of all chromosomes (Waminal et al., 2021). In addition, this study also revealed that StoTR01_86 had undergone expansion, relocating from IGS region of the 45S rDNA to the 5S rDNA in S. hirsuta and S. sophera. The underlying mechanism governing this expansion and movement of the repetitive DNA element StoTR01_86 likely involves transpositional events, which are molecular processes enabling specific DNA sequences, including repetitive elements, to mobilize within a genome. These findings underscore the dynamic nature of plant genomes, the potential influence of repetitive elements on genome evolution, and the intriguing role of StoTR01_86 in shaping the genetic landscape of Senna species.
In our previous investigation, Ta et al. (2021) reported the co-localization of StoTR05_180 with Sto_Tel at subtelomeric regions in most nine studied species, except for S. sulfurea, which exhibited pericentromeric signals across all chromosomes, a similar pattern in S. tora, as detailed in Table 1. Our current study also detected StoTR05_180 in the pericentromeric region of nine chromosomes in S. angulata. This intriguing pattern suggests that the transposition of these TR loci from subtelomeric to pericentromeric regions may have occurred across multiple species, including S. angulata, S. sulfurea, and S. tora. We propose that this transposition event may have involved the ancestral karyotypes of these species. However, it is also plausible that this transposition event occurred through relatively random chromosomal rearrangement events. One potential mechanism for such concerted transposition of tandem repeat arrays is chromoplexy, a complex process involving extensive chromosomal rearrangements across multiple chromosomes, as previously described (Comai and Tan, 2019; Pellestor and Gatinois, 2020). Notably, microhomologies between telomeric and pericentromeric regions make these regions susceptible to chromosomal inversions (He et al., 2013).
It is noteworthy that certain evolutionary events have occurred in the case of S. angulata, S. sulfurea, and S. tora. Specifically, these species have experienced the loss of subtelomeric StoTR05_180 loci while concurrently amplifying pericentromeric loci. In the case of S. tora, an intriguing development has occurred wherein a novel centromeric repeat, StoTR03_178, has emerged. It is highly plausible that StoTR03_178 may have evolved from StoTR05_180 (Waminal et al., 2021). The disruption of the epigenetic landscape immediately following chromosomal rearrangements appears to have facilitated the transformation of StoTR05_180 into a novel centromeric repeat. In S. tora, this transformation may have influenced the positioning and stabilization of StoTR03_178 variants as centromeric elements. The abundance of StoTR03_178 copies relative to StoTR05_180, coupled with its centromeric localization, indicated a notable shift in centromeric repeat preference towards StoTR03_178. This shift likely resulted from restoring proper meiotic pairing following genomic perturbations (Ma and Gustafson, 2005; Schubert and Vu, 2016). Notably, in this study, similar dynamics were also observed in S. angulata. This may support the idea that S. angulata and S. tora underwent comparable evolutionary events involving StoTR03_178 and StoTR05_180 during the development of dysploid karyotypes.
Subrepeat elements located within the IGS play a crucial role in shaping the dynamics of both the IGS itself and the 45S rDNA, thereby influencing genomic variability (Jo et al., 2011; Mirete et al., 2013; Havlová et al., 2016; Ma et al., 2020). The rearrangement and excision of IGS repeat elements introduce variability in IGS length across species, even within the same taxon (Krawczyk et al., 2017). Waminal et al. (2021) previously reported that StoIGS_463 is a duplicated 463 bp sequence and two StoIGS_463 copies were located in the intergenic spacer (IGS) region of S. tora 45S rDNA. However, our recent research revealed that StoIGS_463 was found exclusively in S. sophera but adjacent to 5S rDNA, not 45S rDNA, as observed in S. tora. Intriguingly, StoIGS_463 was also detected in subtelomeric or interstitial chromosomal regions of several other species, including S. alata, S. candolleana, S. corymbosa, and S. floribunda (Ta et al., 2021). This finding suggests that the IGS segment has undergone relocation and amplification within alternative chromosomal regions in these species, departing from its original position at the NOR site, as observed in S. tora (Waminal et al., 2021). These IGS transposable elements exhibit characteristics like transposons, migrating in and out of the 45S rDNA IGS and experiencing amplification within diverse chromosomal locations (Almeida et al., 2012; Waminal et al., 2021). However, the precise mechanisms governing this translocation remain unclear. Combining with our previous research, we propose that the 45S rDNA IGS may act as a carrier during chromosomal rearrangements, occasionally anchoring transposed TR fragments within a taxonomic group and signify the recent involvement of IGS in chromosomal rearrangements (Ta et al., 2021).
Telomeric repeats are typically found at the ends of chromosomes in most eukaryotes. They play an essential role in preventing chromosomal damage (Fuchs et al., 1995; Muraki et al., 2012). Nevertheless, interstitial telomeric repeats (ITRs) are also known to occur in some animal and plant species; however, their size, number, and distribution vary within and between species (He et al., 2013; Sousa and Renner, 2015; Souza et al., 2016; Nguyen et al., 2021). In this study, four of the seven Senna species, namely, S. angulata, S. hirsuta, S. pallida, and S. sophera showed ITR signals and chromosomal rearrangements involving Sto_Tel. This suggests that these species shared a common ancestor that underwent these rearrangements. The absence of ITR signals in the other species may be attributed to the repeat loci being fixed and reduced, making them challenging to detect. ITRs in plants are considered cytological landmarks of chromosomal rearrangements, which could result from various mechanisms, such as ancestral chromosomal fusion events, translocation, inversion, and equivocal dispersion of telomeric DNA (Murat et al., 2010; Sousa and Renner, 2015; Wang et al., 2015; Rosato et al., 2018; Maravilla et al., 2021). ITRs in the (peri)centromeric regions may be remnants of past end-to-end fusion between non-homologous chromosomes, leading to descending dysploidy in S. angulata. Telomeric sequences may also be amplified through mechanisms such as those involved in the genomic evolutionary dynamics of satellite DNA (Charlesworth et al., 1994; Cohen et al., 2008; He et al., 2013; Sousa et al., 2014). Moreover, the DNA double-strand break repair mechanisms may also contribute to the presence of short stretches of ITRs (Lin and Yan, 2008). Furthermore, the mechanisms mentioned are not mutually exclusive and may act together in the evolutionary turnover of plant ITRs.
The observed data indicated that genome size was not directly affected by the number of chromosomes across the examined species. This might underscore the multifaceted nature of genome organization, influenced by factors such as gene density, non-coding regions, and evolutionary events like chromosome fusions and fissions. Notably, species with fewer chromosomes, such as S. angulata, can have a larger genome size attributed to more giant chromosomes or more extensive non-coding regions. Similarly, species like S. artemisioides nothosubsp. sturtii, despite having more chromosomes, exhibit a genome size comparable to those with fewer chromosomes. The abundant amount of TRs in S. angulata may be the reason for the larger genome size of this species compared to other studied species. These observations highlight the importance of delving deeper into the genomic architecture rather than relying solely on chromosome number or genome size as proxies for genetic complexity or evolutionary adaptation.
Finally, when examining the studied TRs, it becomes evident that StoTR01_86 stands out as a relatively conserved marker in most Senna species. In contrast, StoTR04_55 exhibits species specificity, exclusively manifesting a distinct signal within S. tora. The remaining TRs display varying degrees of presence and expression patterns among different species, differing from those observed in S. tora. These findings vividly illustrate the dynamic role of TRs in the speciation and evolutionary processes within the Senna genus. Remarkably, TRs appear more abundant in species with dysploid karyotypes, such as S. angulata and S. tora, with signals concentrated predominantly in (peri)centromeric regions, which serve as hotpots for chromosomal rearrangements (Schubert and Lysak, 2011; Pellestor and Gatinois, 2018; Rosato et al., 2018; Hartley and O’neill, 2019). Interchromosomal rearrangements, encompassing arm translocations, end-to-end translocations, and nested chromosome insertions, could give rise to dysploid species with reduced chromosome numbers within these lineages (Rousselet et al., 2000; Mandáková and Lysak, 2018). Consequently, these observations further substantiate the critical role of TRs as drivers of dysploidy-mediated karyotype evolution and the speciation process.
5 Conclusion
In conclusion, TRs are essential for plant genomes, and they affect genome organization and evolution. We observed a combination of shared and independent evolutionary patterns across the studied species, revealing the dynamics of the TRs and providing a better understanding of the evolutionary relationships among these species. Additionally, this study reveals the dynamics of Senna TRs during speciation. Furthermore, these data offer a cytogenetic perspective on the expansion, contraction, and rearrangement of repeat families within the repeat repository of a lineage. Further studies are required to comprehensively investigate the dynamics of the repeats within the Senna genus. Future studies should encompass the creation of additional TR elements and their applications in a broader array of related species.
Data availability statement
The original contributions presented in the study are publicly available. This data can be found here: NCBI, GCA_014851425.1.
Author contributions
TN: Data curation, Formal analysis, Investigation, Methodology, Software, Visualization, Writing – original draft, Writing – review & editing. BK: Investigation, Writing – review & editing. HK: Conceptualization, Funding acquisition, Project administration, Supervision, Validation, Writing – review & editing.
Funding
The author(s) declare financial support was received for the research, authorship, and/or publication of this article. This study was funded by the National Research Foundation of Korea, grant number: NRF 2020K1A3A1A39112373.
Conflict of interest
The authors declare that the research was conducted in the absence of any commercial or financial relationships that could be construed as a potential conflict of interest.
Publisher’s note
All claims expressed in this article are solely those of the authors and do not necessarily represent those of their affiliated organizations, or those of the publisher, the editors and the reviewers. Any product that may be evaluated in this article, or claim that may be made by its manufacturer, is not guaranteed or endorsed by the publisher.
Supplementary material
The Supplementary Material for this article can be found online at: https://www.frontiersin.org/articles/10.3389/fpls.2023.1288220/full#supplementary-material
References
Aldrich, J. C., Maggert, K. A. (2015). Transgenerational inheritance of diet-induced genome rearrangements in Drosophila. PloS Genet. 11, 1–21. doi: 10.1371/journal.pgen.1005148
Almeida, C., Fonsêca, A., dos Santos, K. G., Mosiolek, M., Pedrosa-Harand, A. (2012). Contrasting evolution of a satellite DNA and its ancestral IGS rDNA in Phaseolus (Fabaceae). Genome. 55, 683–689. doi: 10.1139/g2012-059
Azani, N., Babineau, M., Bailey, C. D., Banks, H., Barbosa, A. R., Pinto, R. B., et al. (2017). A new subfamily classification of the Leguminosae based on a taxonomically comprehensive phylogeny: the Legume phylogeny working group (LPWG). Taxon. 66, 44–77. doi: 10.12705/661.3
Bennett, M. D., Leitch, I. J. (2005). Plant genome size research: A field in focus. Ann. Bot. 95, 1–6. doi: 10.1093/aob/mci001
Biondo, E., Miotto, S. T. S., Schifino-Wittmann, M. T., Castro, B. D. (2005). Cytogenetics and cytotaxonomy of Brazilian species of Senna Mill. (Cassieae-Caesalpinioideae-Leguminosae). Caryologia. 58, 152–163. doi: 10.1080/00087114.2005.10589445
Bourge, M., Brown, S. C., Siljak-Yakovlev, S. (2018). Flow cytometry as tool in plant sciences, with emphasis on genome size and ploidy level assessment. Genet. Applications. 2, 1–12. doi: 10.31383/ga.vol2iss2pp1-12
Bradley Morris, J., Tonnis, B. D., Wang, M. L. (2019). Variability for sennoside A and B concentrations in eight Senna species. Ind. Crops. Prod. 139, 111489. doi: 10.1016/j.indcrop.2019.111489
Chaulagain, B. P., Sakya, S. R. (2002). Inconstancy in chromosome number in some species of Cassia L. found in Nepal. . Nepal J. Sci. Technol. 4, 123–128.
Charlesworth, B., Sniegowski, P., Stephan, W. (1994). The evolutionary dynamics of repetitive DNA in eukaryotes. Nature. 371, 215–220. doi: 10.1038/371215a0
Cohen, S., Houben, A., Segal, D. (2008). Extrachromosomal circular DNA derived from tandemly repeated genomic sequences in plants. Plant J. 53, 1027–1034. doi: 10.1111/j.1365-313X.2007.03394.x
Comai, L., Tan, E. H. (2019). Haploid induction and genome instability. Trends Genet. TIG. 35, 791–803. doi: 10.1016/j.tig.2019.07.005
Cordeiro, J. M. P., Felix, L. P. (2017). Intra- and interspecific karyotypic variations of the genus Senna Mill. (Fabaceae, Caesalpinioideae). Acta Bot. Bras. 32, 128–134. doi: 10.1590/0102-33062017abb0274
de Moraes, R. L., Sassi, F. D., Bertollo, L. A., Marinho, M. M., Viana, P. F., Feldberg, E., et al. (2021). Tracking the evolutionary trends among small-size fishes of the genus Pyrrhulina (Characiforme, Lebiasinidae): new insights from a molecular cytogenetic perspective. Front. Genet. 12. doi: 10.3389/fgene.2021.769984
Doležel, J., Greilhuber, J., Suda, J. (2007). Estimation of nuclear DNA content in plants using flow cytometry. Nat. Protoc. 2, 2233–2244. doi: 10.1038/nprot.2007.310
Elaine, B., Miotto, S. T., Schifino-Wittmann, M. T., De Castro, B. (2005). Cytogenetics and cytotaxonomy of Brazilian species of Senna Mill. (Cassieae–Caesalpinioideae–Leguminosae). Caryologia. 58, 152–163. doi: 10.1080/00087114.2005.10589445
Falistocco, E. (2020). Insight into the chromosome structure of the cultivated tetraploid alfalfa (Medicago sativa subsp. sativa L.) by a combined use of GISH and FISH techniques. Plants. 9, 542. doi: 10.3390/plants9040542
Ferreira, K., Torres, G. A., Sousa, S. M., Santos, A. C. P. (2010). Karyotype, meiotic behavior and pollen features of Senna occidentalis. Biologia. 65, 789–795. doi: 10.2478/s11756-010-0080-0
Fuchs, J., Brandes, A., Schubert, I. (1995). Telomere sequence localization and karyotype evolution in higher plants. Plant Syst. Evol. 196, 227–241. doi: 10.1007/BF00982962
Fukui, K., Nakayama, S., Ohmido, N., Yoshiaki, H., Yamabe, M. (1998). Quantitative karyotyping of three diploid Brassica species by imaging methods and localization of 45s rDNA loci on the identified chromosomes. Theor. Appl. Genet. 96, 325–330. doi: 10.1007/s001220050744
Freyman, W. A., Höhna, S. (2018). Cladogenetic and anagenetic models of chromosome number evolution: a Bayesian model averaging approach. Syst. Biol. 67, 195–215. doi: 10.1093/sysbio/syx065
Garrido-Ramos, M. A. (2017). Satellite DNA: an evolving topic. Genes. 8, 230. doi: 10.3390/genes8090230
Guerra, M. (2008). Chromosome numbers in plant cytotaxonomy: concepts and implications. Cytogenet. Genome Res. 120, 339–350. doi: 10.1159/000121083
Hartley, G., O’neill, R. J. (2019). Centromere repeats: hidden gems of the genome. Genes. 10, 223. doi: 10.3390/genes10030223
Havlová, K., Dvořáčková, M., Peiro, R., Abia, D., Mozgová, I., Vansáčová, L., et al. (2016). Variation of 45S rDNA intergenic spacers in Arabidopsis thaliana. Plant Mol. Biol. 92, 457–471. doi: 10.1007/S11103-016-0524-1
He, L., Liu, J., Torres, G. A., Zhang, H., Jiang, J., Xie, C. (2013). Interstitial telomeric repeats are enriched in the centromeres of chromosomes in Solanum species. Chromosome. Res. 21, 5–13. doi: 10.1007/s10577-012-9332-x
Hwang, Y. J., Song, C. M., Younis, A., Kim, C. K., Kang, Y. I., Lim, K. B. (2015). Morphological characterization under different ecological habitats and physical mapping of 5S and 45S rDNA in Lilium distichum with fluorescence in situ hybridization. Rev. Chil. Hist. Nat. 88, 1–13. doi: 10.1186/S40693-015-0037-3
Iovene, M., Wielgus, S. M., Simon, P. W., Buell, C. R., Jiang, J. (2008). Chromatin structure and physical mapping of chromosome 6 of potato and comparative analyses with tomato. Genetics. 180, 1307–1317. doi: 10.1534/genetics.108.093179
Irwin, H. S., Turner, B. L. (1960). Chromosomal Relat. taxonomic considerations genus Cassia. Am. J. Bot. 47, 309–318. doi: 10.1002/j.1537-2197.1960.tb07130.x
Jack, C. V., Cruz, C., Hull, R. M., Keller, M. A., Ralser, M., Houseley, J. (2015). Regulation of ribosomal DNA amplification by the TOR pathway. PNAS. 112, 9674–9679. doi: 10.1073/pnas.1505015112
Jo, S.-H., Park, H.-M., Kim, S.-M., Kim, H. H., Hur, C.-G., Choi, D. (2011). Unraveling the sequence dynamics of the formation of genus-specific satellite DNAs in the family Solanaceae. . Heredity. 106, 876–885. doi: 10.1038/hdy.2010.131
Kang, S. H., Lee, W. H., Lee, C. M., Sim, J. S., Won, S. Y., Han, S. R., et al. (2020a). De novo transcriptome sequence of Senna tora provides insights into anthraquinone biosynthesis. PloS One 15, e0225564. doi: 10.1371/journal.pone.0225564
Kang, S. H., Pandey, R. P., Lee, C. M., Sim, J. S., Jeong, J. T., Choi, B. S., et al. (2020b). Genome-enabled discovery of anthraquinone biosynthesis in Senna tora. Nat. Commun. 11, 1–11. doi: 10.1038/S41467-020-19681-1
Kobayashi, T. (2011). Regulation of ribosomal RNA gene copy number and its role in modulating genome integrity and evolutionary adaptability in yeast. Cell. Mol. Life Sci. 68, 1395–1403. doi: 10.1007/s00018-010-0613-2
Kobayashi, T., Sasaki, M. (2017). Ribosomal DNA stability is supported by many ‘buffer genes’—introduction to the yeast rDNA stability database. FEMS Yeast Res. 17, 1–8. doi: 10.1093/femsyr/fox001
Koo, D.-H., Nam, Y.-W., Choi, D., Bang, J.-W., De Jong, H., Hur, Y. (2010). Molecular cytogenetic mapping of Cucumis sativus and C. melo using highly repetitive DNA sequences. Chromosome Res. 18, 325–336. doi: 10.1007/s10577-010-9116-0
Krawczyk, K., Nobis, M., Nowak, A., Szczecińska, M., Sawicki, J. (2017). Phylogenetic implications of nuclear rRNA IGS variation in Stipa L. (Poaceae). Sci. Rep. 7, 11506. doi: 10.1038/S41598-017-11804-x
Levan, A., Fredga, K., Sandberg, A. A. (1964). Nomenclature for centromeric position on chromosomes. Hereditas. 52, 201–220. doi: 10.1111/j.1601-5223.1964.tb01953.x
Lin, K. W., Yan, J. (2008). Endings in the middle: current knowledge of interstitial telomeric sequences. Mutat. Res. 658, 95–110. doi: 10.1016/j.mrrev.2007.08.006
Louzada, S., Lopes, M., Ferreira, D., Adega, F., Escudeiro, A., Gama-Carvalho, M., et al. (2020). Decoding the role of satellite DNA in genome architecture and plasticity - an evolutionary and clinical affair. Genes. 11, 72. doi: 10.3390/genes11010072
Ma, Y., Liu, T., Liu, B., Gao, L., Chen, W. (2020). Population genetic structures of Puccinia triticina in five provinces of China. Eur. J. Plant Pathol. 156, 1135–1145. doi: 10.1007/S10658-020-01956-4
Ma, X.-F., Gustafson, J. P. (2005). Genome evolution of allopolyploids: a process of cytological and genetic diploidization. Cytogenet. Genome Res. 109, 236–249. doi: 10.1159/000082406
Mahelka, V., Kopecký, D., Baum, B. R. (2013). Contrasting patterns of evolution of 45S and 5S rDNA families uncover new aspects in the genome constitution of the agronomically important grass Thinopyrum intermedium (Triticeae). Mol. Biol. Evolution. 30, 2065–2086. doi: 10.1093/molbev/mst106
Maluszynska, J., Heslop-Harrison, J. (1993). Physical mapping of rDNA loci in Brassica species. Genome 36, 774–781. doi: 10.1139/g93-102
Mandáková, T., Joly, S., Krzywinski, M., Mummenhoff, K., Lysaka, M. A. (2010). Fast diploidization in close mesopolyploid relatives of Arabidopsis. Plant Cell. 22, 2277–2290. doi: 10.1105/tpc.110.074526
Mandáková, T., Lysak, M. A. (2018). Post-polyploid diploidization and diversification through dysploid changes. Curr. Opin. Plant Biol. 42, 55–65. doi: 10.1016/j.pbi.2018.03.001
Mandáková, T., Pouch, M., Brock, J. R., Al-Shehbaz, I. A., Lysak, M. A. (2019). Origin and evolution of diploid and allopolyploid Camelina genomes were accompanied by chromosome shattering. Plant Cell. 31, 2596–2612. doi: 10.1105/tpc.19.00366
Maravilla, A. J., Rosato, M., Rosselló, J. A. (2021). Interstitial telomeric-like repeats (ITR) in seed plants as assessed by molecular cytogenetic techniques: a review. Plants. 10, 2541. doi: 10.3390/plants10112541
Mirete, S., Patiño, B., Jurado, M., Vázquez, C., González-Jaén, M. T. (2013). Structural variation and dynamics of the nuclear ribosomal intergenic spacer region in key members of the Gibberella fujikuroi species complex. Genome. 56, 205–213. doi: 10.1139/gen-2013-0008
Muraki, K., Nyhan, K., Han, L., Murnane, J. P. (2012). Mechanisms of telomere loss and their consequences for chromosome instability. Front. Oncol. 2. doi: 10.3389/fonc.2012.00135
Murat, F., Xu, J. H., Tannier, E., Abrouk, M., Guilhot, N., Pont, C., et al. (2010). Ancestral grass karyotype reconstruction unravels new mechanisms of genome shuffling as a source of plant evolution. Genome. Res. 20, 1545–1557. doi: 10.1101/gr.109744.110
Nguyen, T. H., Park, S. J., Kang, B. Y., Kim, H. H.. (2023). Comparative triple-color FISH mapping and genome size advances understanding of the cytogenetic diversity in wild Solanum species. Hortic. Environ. Biotechnol. 64, 811–817. doi: 10.1007/s13580-023-00522-1
Nguyen, T. H., Waminal, N. E., Lee, D. S., Pellerin, R. J., Ta, T. D., Campomayor, N. B., et al. (2021). Comparative triple-color FISH mapping in eleven Senna species using rDNA and telomeric repeat probes. Hortic. Environ. Biotechnol. 62, 927–935. doi: 10.1007/S13580-021-00364-9
Novák, P., Robledillo, L.Á., Koblížková, A., Vrbová, I., Neumann, P., Macas, J. (2017). TAREAN: a computational tool for identification and characterization of satellite DNA from unassembled short reads. Nucleic. Acids Res. 45, e111. doi: 10.1093/nar/gkx257
Oladeji, O. S., Adelowo, F. E., Oluyori, A. P. (2021). The genus Senna (Fabaceae): a review on its traditional uses, botany, phytochemistry, pharmacology and toxicology. S. Afr. J. Bot. 138, 1–32. doi: 10.1016/j.sajb.2020.11.017
Oliur Rahman, M., Rahman, Z., Begum, A. (2013). Numerical taxonomy of the genus Senna Mill. from Bangladesh. Bangladesh J. Plant Taxon. 20, 77–83. doi: 10.3329/bjpt.v20i1.15467
Ongchai, S., Chokchaitaweesuk, C., Kongdang, P., Chomdej, S., Buddhachat, K. (2019). In vitro chondroprotective potential of Senna alata and Senna tora in porcine cartilage explants and their species differentiation by DNA barcoding-high resolution melting (Bar-HRM) analysis. PloS One 14, e0215664. doi: 10.1371/journal.pone.0215664
Paredes, S., Branco, A. T., Hartl, D. L., Maggert, K. A., Lemos, B. (2011). Ribosomal DNA deletions modulate genome-wide gene expression: “rDNA-sensitive” genes natural variation. PloS Genet. 7, 1–10. doi: 10.1371/journal.pgen.1001376
Pellestor, F., Gatinois, V. (2018). Chromoanasynthesis: another way for the formation of complex chromosomal abnormalities in human reproduction. Hum. Reprod. 33, 1381–1387. doi: 10.1093/humrep/dey231
Pellestor, F., Gatinois, V. (2020). Chromoanagenesis: a piece of the macroevolution scenario. Mol. Cytogenet. 13, 3. doi: 10.1186/s13039-020-0470-0
Perumal, S., Waminal, N. E., Lee, J., Lee, J., Choi, B. S., Kim, H. H., et al. (2017). Elucidating the major hidden genomic components of the A, C, and AC genomes and their influence on Brassica evolution. Sci. Rep. 7, 17986. doi: 10.1038/S41598-017-18048-9
Puri, B. K. (2018). Editorial: the potential medicinal uses of Cassia tora Linn leaf and seed extracts. Rev. Recent. Clin. Trials. 13, 3–4. doi: 10.2174/157488711301180131145359
Resende, K., Prado, C., Davide, L., Torrea, G. (2014). Polyploidy and apomixis in accessions of Senna rugosa (G.Don) H.S. Irwin & Barneby. Turk. J. Biol. 38, 510–515. doi: 10.3906/biy-1312-66
Roa, F., Guerra, M. (2012). Distribution of 45S rDNA sites in chromosomes of plants: structural and evolutionary implications. BMC. Evol. Biol. 12, 225. doi: 10.1186/1471-2148-12-225
Rosato, M., Álvarez, I., Feliner, G. N., Rosselló, J. A. (2018). Inter- and intraspecific hypervariability in interstitial telomeric-like repeats (TTTAGGG)n in Anacyclus (Asteraceae). Ann. Bot. 122, 387–395. doi: 10.1093/aob/mcy079
Rousselet, J., Monti, L., Auger-Rozenberg, M. A., Parker, J. S., Lemeunier, F. (2000). Chromosome fission associated with growth of ribosomal DNA in Neodiprion abietis (Hymenoptera: Diprionidae). Proceed. Biol. Sci. 267, 1819–1823. doi: 10.1098/rspb.2000.1216
Salim, D., Bradford, W. D., Freeland, A., Cady, G., Wang, J., Pruitt, S. C., et al. (2017). DNA replication stress restricts ribosomal DNA copy number. PloS Genet. 13, 1–20. doi: 10.1371/journal.pgen.1007006
Salim, D., Gerton, J. L. (2019). Ribosomal DNA instability and genome adaptability. Chromosome Res. 27, 73–87. doi: 10.1007/s10577-018-9599-7
Sassi, F. M. C., Oliveira, E. A., Bertollo, L. A. C., Nirchio, M., Hatanaka, T., Marinho, M. M. F., et al. (2019). Chromosomal evolution and evolutionary relationships of Lebiasina species (Characiformes, Lebiasinidae). Int. J. Mol. Sci. 20, 2944. doi: 10.3390/ijms20122944
Schubert, I., Lysak, M. A. (2011). Interpretation of karyotype evolution should consider chromosome structural constraints. Trends. Genet. 27, 207–216. doi: 10.1016/j.tig.2011.03.004
Schubert, I., Vu, G. T. H. (2016). Genome stability and evolution: attempting a holistic view. Trends Plant Sci. 21, 749–757. doi: 10.1016/j.tplants.2016.06.003
Sousa, A., Cusimano, N., Renner, S. S. (2014). Combining FISH and model-based predictions to understand chromosome evolution in Typhonium (Araceae). Ann. Bot. 113, 669–680. doi: 10.1093/aob/mct302
Sousa, A., Renner, S. S. (2015). Interstitial telomere-like repeats in the monocot family Araceae. Bot. J. Linn. Soc 177, 15–26. doi: 10.1111/boj.12231
Souza, G., Vanzela, A. L. L., Crosa, O., Guerra, M. (2016). Interstitial telomeric sites and Robertsonian translocations in species of Ipheion and Nothoscordum (Amaryllidaceae). Genetica. 144, 157–166. doi: 10.1007/s10709-016-9886-1
Ta, T. D., Waminal, N. E., Nguyen, T. H., Pellerin, R. J., Kim, H. H. (2021). Comparative FISH analysis of Senna tora tandem repeats revealed insights into the chromosome dynamics in Senna. Genes Genomics 43, 237–249. doi: 10.1007/s13258-021-01051-w
Volkov, R. A., Panchuk, I. I., Borisjuk, N. V., Hosiawa-Baranska, M., Maluszynska, J., Hemleben, V., et al. (2017). Evolutional dynamics of 45S and 5S ribosomal DNA in ancient allohexaploid Atropa belladonna. BMC Plant Biol. 17, 21. doi: 10.1186/s12870-017-0978-6
Waminal, N. E., Kim, H. H. (2012). Dual-color FISH karyotype and rDNA distribution analyses on four Cucurbitaceae species. Hortic. Environ. Biotechnol. 53, 49–56. doi: 10.1007/s13580-012-0105-4
Waminal, N. E., Pellerin, R. J., Kang, S. H., Kim, H. H. (2021). Chromosomal mapping of tandem repeats revealed massive chromosomal rearrangements and insights into Senna tora dysploidy. Front. Plant Sci. 12. doi: 10.3389/fpls.2021.629898
Waminal, N. E., Pellerin, R. J., Kim, N. S., Jayakodi, M., Park, J. Y., Yang, T. J., et al. (2018). Rapid and efficient FISH using pre-labeled oligomer probes. Sci. Rep. 8, 8224. doi: 10.1038/s41598-018-26667-z
Wang, X., Jin, D., Wang, Z., Guo, H., Zhang, L., Wang, L., et al. (2015). Telomere-centric genome repatterning determines recurring chromosome number reductions during the evolution of eukaryotes. New Phytol. 205, 378–389. doi: 10.1111/nph.12985
Watanabe, K., King, R. M., Yahara, T., Ito, M., Yokoyama, J., Suzuki, T., et al. (1995). Chromosomal cytology and evolution in Eupatorieae (Asteraceae). Ann. Mo Bot. Gard. 82, 581–592. doi: 10.2307/2399838
Keywords: chromosomal rearrangement, FISH, genome dynamic, Senna, tandem repeat
Citation: Nguyen TH, Kang BY and Kim HH (2023) Chromosomal dynamics in Senna: comparative PLOP–FISH analysis of tandem repeats and flow cytometric nuclear genome size estimations. Front. Plant Sci. 14:1288220. doi: 10.3389/fpls.2023.1288220
Received: 05 September 2023; Accepted: 08 November 2023;
Published: 14 December 2023.
Edited by:
Wellington Ronildo Clarindo, Universidade Federal de Viçosa, BrazilReviewed by:
Renata Carvalho, Universidad de la República, UruguayMariana Cansian Sattler, Universidade Federal de Viçosa, Brazil
Ana Christina Brasileiro-Vidal, Federal University of Pernambuco, Brazil
Copyright © 2023 Nguyen, Kang and Kim. This is an open-access article distributed under the terms of the Creative Commons Attribution License (CC BY). The use, distribution or reproduction in other forums is permitted, provided the original author(s) and the copyright owner(s) are credited and that the original publication in this journal is cited, in accordance with accepted academic practice. No use, distribution or reproduction is permitted which does not comply with these terms.
*Correspondence: Hyun Hee Kim, a2ltaGhAc3l1LmFjLmty