- 1Institute of Grass Research, Heilongjiang Academy of Agricultural Sciences, Harbin, China
- 2Department of Grassland Science, College of Animal Science and Technology, Northeast Agricultural University, Harbin, China
Low-temperature stress is a major environmental factor that limits the yield, quality, and geographical distribution of forage crops and restricts the development of the forage industry. As a core component of plant circadian clocks, Late Elongated Hypocotyl (LHY) plays a crucial role in regulating plant rhythms and responses to abiotic stress. However, the molecular mechanism by which LHY regulates the cold tolerance of alfalfa has not been reported. In this study, MsLHY, which is 2,235 bp in length and encodes 744 amino acids, was isolated from alfalfa. MsLHY was highly expressed in roots and stems and was significantly induced by low temperature. Transgenic MsLHY-overexpressing (OE) and RNAi alfalfa plants were obtained via Agrobacterium-mediated transformation. Under low-temperature stress, OE plants presented reduced reactive oxygen species accumulation and more osmotic regulatory substances, as well as greater antioxidant enzyme activity, to combat cold stress. Conversely, the RNAi plants presented trends opposite those of the OE plants. Furthermore, under cold stress, the overexpression of MsLHY upregulated the expression of the cold-responsive genes MsICE1, MsCBF1, MsCOR15A, and MsCML10, as well as the expression of the antioxidant-synthesizing genes MsSOD1 and MsCAT1, thereby increasing the cold tolerance of transgenic alfalfa. These results suggest that MsLHY plays an important role in increasing the cold tolerance of alfalfa.
1 Introduction
Abiotic stress, especially cold stress, has major effects on plant growth and development (Mickelbart et al., 2015; Ritonga and Chen, 2020), and the agricultural economy suffers losses of hundreds of billions of yuan every year due to low temperatures (Li and Christersson, 1992). Low-temperature stress results in cold damage at low temperatures above 0°C and freezing damage at low temperatures below 0°C (Chen et al., 2022). After sensing low-temperature signals, plants resist low-temperature stress through a series of molecular mechanisms and physiological regulation. C-repeat-binding factors (CBFs), inducers of CBF expression (ICEs) and cold-regulated genes (CORs) play key roles in plant cold adaptation (Lee and Thomashow, 2012; Ding et al., 2019). These genes form the ICE-CBF-COR pathway, which alleviates plant damage caused by low-temperature stress (Thomashow, 1999; Shu et al., 2017; Wang et al., 2017; Guo et al., 2019), and the active elements of the ICE-CBF-COR pathway have been identified in rice and other crop species (Bremer et al., 2017). In addition, the activation of protective enzymes and metabolites related to cold resistance; the synthesis of protective proteins and metabolism-related complexes; and the maintenance of a new energy balance, metabolite balance and redox balance are crucial for increasing low-temperature tolerance in plants (Murata et al., 2007; Grabelnych et al., 2014; Shi et al., 2018; Ambroise et al., 2020).
Late Elongated Hypocotyl (LHY) is a key circadian clock gene in Arabidopsis thaliana that encodes a protein belonging to the MYB family of transcription factors that play a central role in regulating the circadian rhythm of plants, affecting their growth, flowering time, and response to environmental changes (Mi-Jeong et al., 2016; Adams et al., 2018). The LHY protein contains several functional domains, including the SANT, homeodomain-like, Myb, and DNA-binding domains. These domains give LHY the ability to bind to DNA, thereby regulating the expression of specific genes (Atamian and Harmer, 2016). LHY, together with Circadian Clock Associated 1 (CCA1) and Timing Of Cab 1 (TOC1), constitute the core oscillators of the plant biological clock (Hung et al., 2019; Su et al., 2021). The expression of Pseudo-Response Regulator (PRR) family genes is inhibited by LHY and CCA1, and these genes in turn negatively regulate the expression of CCA1 and LHY, forming a complex regulatory network (Farre and Liu, 2013). The LHY gene also accelerates flowering in plants under continuous light conditions by promoting the expression of the Flowering Locus T (FT) gene (Liu et al., 2024).
In addition, the LHY gene plays an important role in the abiotic stress response. This gene influences drought tolerance in plants by regulating the response of the plant hormone abscisic acid (ABA) (Adams et al., 2018). Studies have shown that under drought conditions, the expression of the LHY gene increases, directly inhibiting the target genes of multiple ABA signaling pathways and thus affecting the drought response of plants (Wang et al., 2020). Moreover, the LHY gene is associated with water use efficiency (WUE) in plants, regulating water retention and use by influencing ABA biosynthesis and signaling (Xu et al., 2022). Studies have also shown that LHY transcription factors are involved in the regulation of the plant response to low temperature, especially during vernalization. LHY together with CCA1 activates VIN3 transcription through the vernalization reaction cis-element, which is a key step in the acquisition of long-term cold-mediated flowering ability (Kyung et al., 2022). The expression of the LHY gene also changed under low-temperature stress, and the cold-induced expression of the DREB1 gene in the cca1 lhy double mutant A. thaliana significantly decreased (Dong et al., 2011). EEs are present in the promoter region of DREB1 and many cold-induced genes, and CCA1 LHY can bind EEs to inhibit the expression of evening genes (Harmer and Kay, 2005). These findings reveal the regulatory mechanism of the LHY gene during plant adaptation to low-temperature environments and provide an important molecular basis for further research and improvement of plant cold tolerance.
Alfalfa (Medicago sativa L.), known as the “king of grass”, is an excellent perennial grass of the Leguminosae family, with a high protein content and high nutritional value (Annicchiarico et al., 2015). Its strong adaptability in various soil and climate conditions, is an indispensable component of animal husbandry (Wang et al., 2024). In northern China, winters are cold, and extremely low temperatures occur frequently, during late spring and early fall causing major losses in the growth and production of alfalfa; thus, low temperatures are among the major environmental factors restricting the industrialization of alfalfa in northern China (Liu et al., 2006). Therefore, studying the physiological and molecular response mechanisms of alfalfa under low-temperature stress, increasing its adaptability and productivity in cold areas, reducing economic losses caused by low temperature, promoting the sustainable development of animal husbandry, and providing a scientific basis for breeding cold-resistant varieties are highly important.
The LHY-like protein associated with cold stress was identified in our previous transcriptomic data, but the function of LHY and its role in cold tolerance have not been fully elucidated. In this study, we cloned MsLHY and examined its relative expression level under abiotic stress to determine how it is expressed under abiotic stress and found that MsLHY is strongly induced by low temperature. Compared with RNAi plants, MsLHY-overexpressing transgenic alfalfa presented increased cold tolerance at 4°C and -5°C. Similarly, the expression of cold stress response genes in overexpressing plants was also significantly induced to increase the cold tolerance of alfalfa. In this study, the function and regulatory mechanism of the LHY gene in the cold tolerance of alfalfa was explored to provide a knowledge basis for the breeding and genetic improvement of cold-tolerant varieties of alfalfa.
2 Materials and methods
2.1 Plant materials and stress treatments
Alfalfa (M. sativa L. cv. Zhongmu No. 1) seeds were treated with 75% ethanol solution for 60 s, followed by 10% NaClO solution treatment for 10 min, washed with sterile water 5 times and placed on Petri dishes with wet filter paper. After 5 days of germination, healthy seedlings with uniform growth were selected and moved to a cavity dish filled with soil and vermiculite at a ratio of 1:1, placed in an incubator under a 16/8 h light/dark photoperiod (25/20°C) with 60% relative humidity, and watered with alfalfa optimized nutrient solution every 2 days (Zhang, 2014). When reaching the five-leaf stage, the seedlings were subjected to osmotic stress to simulate drought (20% PEG-6000), salt (150 mM NaCl), saline–alkaline (150 mM NaHCO3) or low-temperature (4°C) conditions. Samples were collected from above and below ground at 0 h, 2 h, 4 h, 8 h, 12 h, 24 h and 48 h, with 3 biological replicates per treatment. The samples were stored in a labeled centrifuge tube, rapidly frozen in liquid nitrogen and stored at -80°C.
2.2 Full-length CDS cloning of MsLHY
The total RNA of alfalfa was extracted using an RNA extraction kit (Vazyme, Nanjing, China) according to the manufacturer’s instructions, and the first strand of cDNA was synthesized according to the manufacturer’s instructions (Vazyme, Nanjing, China). Specific gene cloning primers (Supplementary Table S1) were designed using the reference sequence of the genome (AES82836.2) of Medicago truncatula, and 50 μL polymerase chain reaction (PCR) was used to amplify the gene fragment using alfalfa cDNA as a template. The PCR amplification program was as follows: 95°C for 3 min; 35 cycles of 95°C for 10 s, 60°C for 20 s, and 72°C for 40 s; and 72°C for 5 min (Vazyme, Nanjing, China). The fragment length was verified via agarose gel electrophoresis. The DNA fragments were purified using a product purification kit (Vazyme, Nanjing, China), the purified product was ligated into the pCE2 TA/Blunt-Zero Cloning vector using a 5-min TA/Blunt-Zero Cloning Kit (Vazyme, Nanjing, China), and the recombinant product was introduced into E. coli DH5α (Weidi, Shanghai, China).
2.3 Bioinformatic analysis of MsLHY
The amino acid sequence was obtained via transcriptomic approaches. The MsLHY sequence was obtained from SnapGene. The homologous sequences of MsLHY were identified from NCBI (https://www.ncbi.nlm.nih.gov/), multiple sequence comparisons were performed using DNAMAN 6.0.3.99, a phylogenetic tree was constructed using MEGA 7.0, and the phylogenetic tree was visualized using Evolview (https://www.evolgenius.info/evolview/#/treeview). Domains in the amino acid sequence encoded by MsLHY was predicted using SMART (http://smart.embl.de/smart/set_mode.cgi?GENOMIC=1), and the physicochemical properties of the MsLHY protein were analyzed using ExPASy (https://web.expasy.org/protparam/). PredictProtein (https://predictprotein.org/) and SWISS-MODEL (https://swissmodel.expasy.org/) were used to predict the secondary structure of the MsLHY protein, and a tertiary structure model was constructed. NetPhos 3.1 (https://services.healthtech.dtu.dk/services/NetPhos-3.1/), ProtScale (https://web.expasy.org/protscale/), TMHMM Server v.2.0 (http://www.cbs.dtu.dk/services/TMHMM-2.0/) and SignalP 4.1 (http://www.cbs.dtu.dk/services/SignalP-4.1/) were used to analyze and predict the phosphorylation sites, hydrophilicity, transmembrane domains and signal peptides of the MsLHY protein.
2.4 Interacting protein prediction
After the MsLHY protein sequence was imported into the STRING (https://cn.string-db.org/) database, the proteins that may interact with MsLHY were analyzed and predicted.
2.5 Gene expression analysis
Quantitative real-time PCR (qRT–PCR) primers (Supplementary Table S1) for MsLHY were designed using NCBI Primer BLAST (http://www.ncbi.nlm.nih.gov/tools/primer-blast/). The alfalfa GAPDH gene was used as the internal housekeeping gene for qRT–PCR (q225MX-400, Kubotech, China) to determine the expression levels and tissue-specific expression levels of MsLHY under different stresses. The qRT–PCR amplification program was as follows: 95°C for 30 s; 40 cycles of 95°C for 10 s, 60°C for 30 s, and 95°C for 15 s; 60°C for 1 min; and 95°C for 15 s. The 2−ΔΔCT comparative method was used to determine how each gene was expressed (Livak and Schmittgen, 2001).
2.6 Generation of MsLHY transgenic plants
The PEG100 vector (Biorun, Wuhan, China) was digested with BamHI and XbaI, and the target gene was inserted into the linearized vector to obtain the PEG100-MsLHY-OE recombinant overexpression vector. A PEG100-MsLHY-RNAi recombinant RNAi vector was obtained by inserting 200 bp MsLHY gene-specific sequences and loop structure into the PEG100 vector cut by the BamHI and XbaI enzymes by Golden Gate method. After two recombinant vectors were transformed into the Agrobacterium tumefaciens strain EHA105 (Weidi, Shanghai, China), alfalfa was transformed via the Agrobacterium-mediated leaf disc method (Ke et al., 1998). Since the PEG100 vector has a Bar resistance gene, 1 mg·L-1 Basta was used to screen for transformed plants. The transformants were transplanted into the soil, and the leaves of the screened plants were assessed using Bar test paper (Biorun, Wuhan, China). RNA was extracted and reverse-transcribed into cDNA. PCR detection was subsequently performed using Bar gene-specific primers (Supplementary Table S1). Specific qPCR primers for MsLHY were designed, and the relative expression level of MsLHY in the transgenic plants was determined with the alfalfa GAPDH gene as the internal housekeeping gene (Supplementary Table S1).
2.7 Cold treatment and determination of the physiological indices of the transgenic plants
WT and transgenic alfalfa were propagated via the asexual cutting propagation method; three plants were planted in a pot of vermiculite and nutrient soil (1:1), cultivated under a 16/8 h light/dark photoperiod (25/20°C) with 60% relative humidity, and watered with alfalfa optimized nutrient solution every 3 days. After five weeks, the pots were placed in an incubator at 4°C and -5°C with 16/8 h day/night and 60% humidity for cold resistance determination. To mimick temperature drop in nature, the incubator was subjected to gradient cooling at 1°C/h. After treatment at 4°C for 7 days and -5°C for 8 h, samples were taken from the aboveground parts of the plants at the same time point in the photoperiod, with 3 biological replicates per treatment. The plants were stored in a marked centrifuge tube, rapidly frozen in liquid nitrogen and stored at -80°C. The relative conductivity was determined according to the methods of Song et al. (2006) with some modifications. Leaves (0.1 g) were incubated in 10 mL of deionized water at 4°C for 12 h. The relative conductivity (C1) of the incubated solution was determined. The sample was then boiled for 15 min, and the conductivity (C2) of the solution was again determined. The relative conductivity was calculated as (C1/C2) × 100%. Superoxide dismutase (SOD) activity, peroxidase (POD) activity, catalase (CAT) activity, soluble protein (SP) content, soluble sugar (SS) content, hydrogen peroxide (H2O2) content, superoxide anion (O2-) content, proline (Pro) content and malondialdehyde (MDA) content were measured using a kit (Comin, Suzhou, China) using living tissue.
2.8 Analysis of expression of stress response genes
The expression of six genes involved in the low-temperature stress response pathway in WT and transgenic alfalfa was determined via qRT–PCR to understand how MsLHY responds to low-temperature stress at the gene level. MsICE1, MsCBF1 and MsCOR15A are the key genes of the cold stress pathway. MsSOD1 and MsCAT encode SOD and CAT, respectively. The MsCML10 gene encodes a member in cold-induced Ca2+ signaling and plays a key role in regulating plant cold tolerance (Yu et al., 2022). The GAPDH gene is an internal housekeeping gene. The sequences of the primers used in this study are listed in Supplementary Table S1.
2.9 Statistical analyses
Statistical analysis of the results was performed via one-way analysis of variance (ANOVA) using SPSS 19, and Duncan’s multiple range test was used to identify significant differences between groups. The means were considered significantly different at p < 0.05.
3 Results
3.1 Characterization and Isolation of MsLHY
We previously identified LHY-like proteins in a transcriptomic study. The full-length cDNA sequence of M. sativa L. cv. Zhongmu No. 1 was used as a template, and the MsLHY gene was subsequently cloned using a specific primer designed with the reference sequence of M. truncatula (AES82836.2). Sequence analysis revealed that MsLHY has an open reading frame of 2,235 bp (Figure 1A) encoding a protein of 744 amino acids with the molecular formula C3501H5565N1015O1155S29. The predicted molecular weight is 81.286 kDa, and the theoretical isoelectric point (pI) is 6.03. SMART domain analysis revealed the SANT domain at amino acids 25-73, indicating that MsLHY is a member of the Myb/SANT family (Supplementary Figure S1A). The secondary structure of the MsLHY protein consists of 17.65% α-helices, 4.41% β-turn and 57.94% random coils. The content of serine (Ser) was the highest in terms of amino acid composition, accounting for 11.2% (Supplementary Figure S1B). The alanine (Ala) content, 8.3%, was second only to the serine content. Ninety-one amino acid residues were negatively charged, and 81 were positively charged. The instability index of the MsLHY protein was 56.68 (greater than 40), indicating that the protein could not exist stably in vitro and was unstable. The fat solubility index was 64.45.
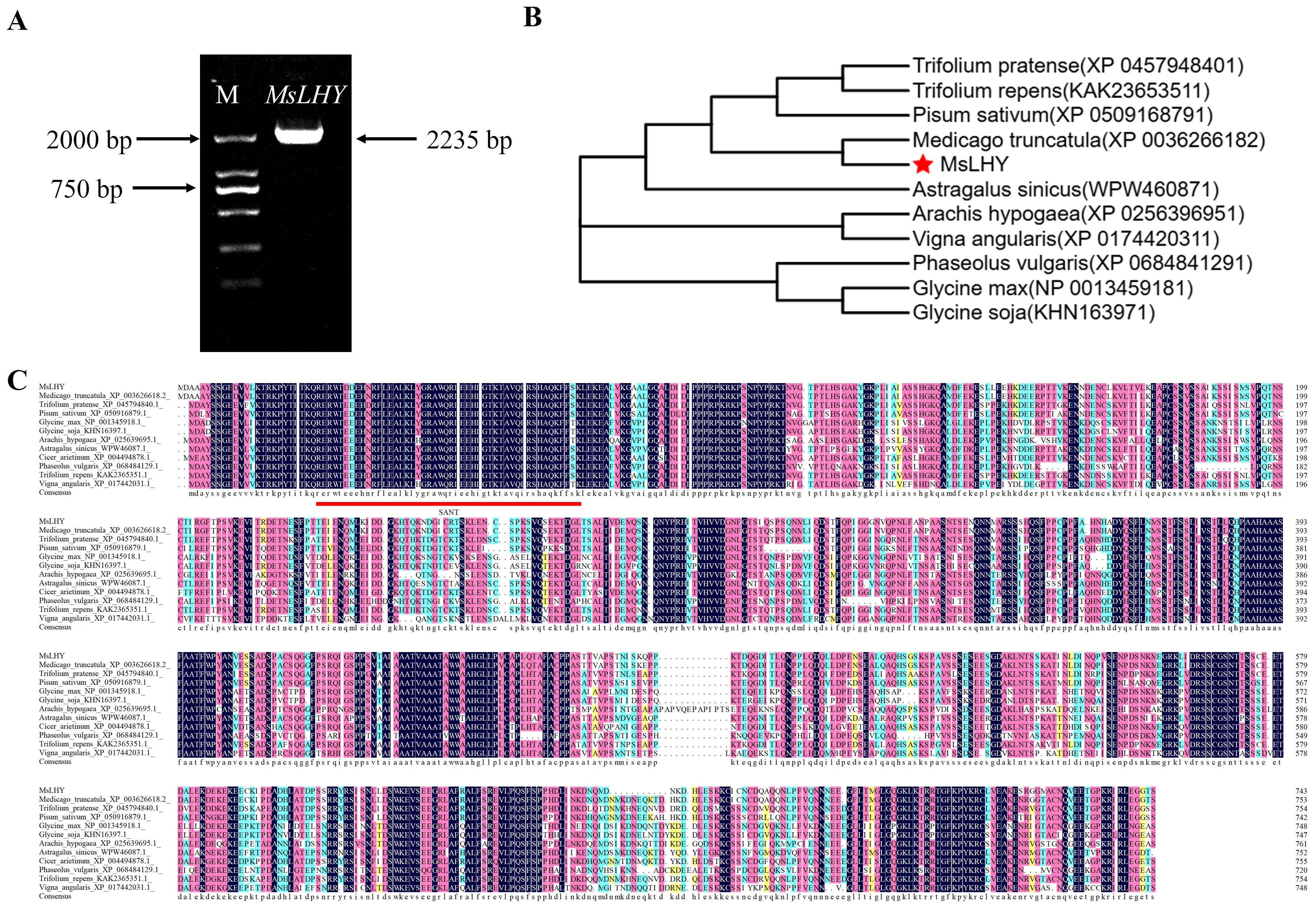
Figure 1. Gene isolation, multiple sequence alignment and phylogenetic tree of MsLHY. (A) Cloning of MsLHY. (B) Phylogenetic tree of MsLHY. (C) Amino acid sequence alignment.
The physicochemical property analysis revealed 94 predicted phosphorylation sites for the MsLHY protein, including 62 serine phosphorylation sites (serine), 2 tyrosine phosphorylation sites (tyrosine), and 30 threonine phosphorylation sites (threonine) (Supplementary Figure S2A). The average hydrophilic value of MsLHY is -0.654, indicating that MsLHY is a hydrophilic protein (Supplementary Figure S2B). The MsLHY protein has no transmembrane domains (Supplementary Figure S2C), no signal peptide splicing sites, and no signal peptide sequences; it is a nonsecretory protein, and it cannot transport proteins (Supplementary Figure S2D).
Multiple sequence comparisons of amino acids performed using DNAMAN revealed that the sequences of M. truncatula (XP_003626618.2), Trifolium pratense (XP_045794840.1), Trifolium repens (KAK2365351.1), Pisum sativum (XP_050916879.1), Cicer arietinum (XP_004494878.1), Astragalus sinicus (WPW46087.1), Glycine soja (KHN16397.1), Glycine max (NP_001345918.1), Phaseolus vulgaris (XP_068484129.1), and Arachis hypogaea (XP_025639695.1) presented 97.35%, 85.77%, 85.64%, 83.87%, 82.85%, 76.96%, 72.88%, 71.73%, 67.60%, and 67.23% homology, respectively, with the SANT domains (Figure 1C). Phylogenetic trees were constructed, revealing that the MsLHY protein has high homology with MtLHY, which has the closest genetic relationship (Figure 1B).
3.2 Prediction of MsLHY-interacting proteins
The proteins that may interact with MsLHY were preliminarily predicted using the STRING database (Figure 2A). A total of 10 possible interacting proteins were predicted, of which 8 were enriched in the rhythmic process, 2 were enriched in the circadian rhythm, 9 were enriched in cellular processes, 10 were enriched in biological processes, 5 were enriched in the phospholipid signal transduction system, and 5 were enriched in the cytokine-activated signaling pathway (Figure 2B). According to our results, the interaction between PRR (G7JRK3_MEDTR) and MsLHY (G7L558_MEDTR) is the most likely interaction.
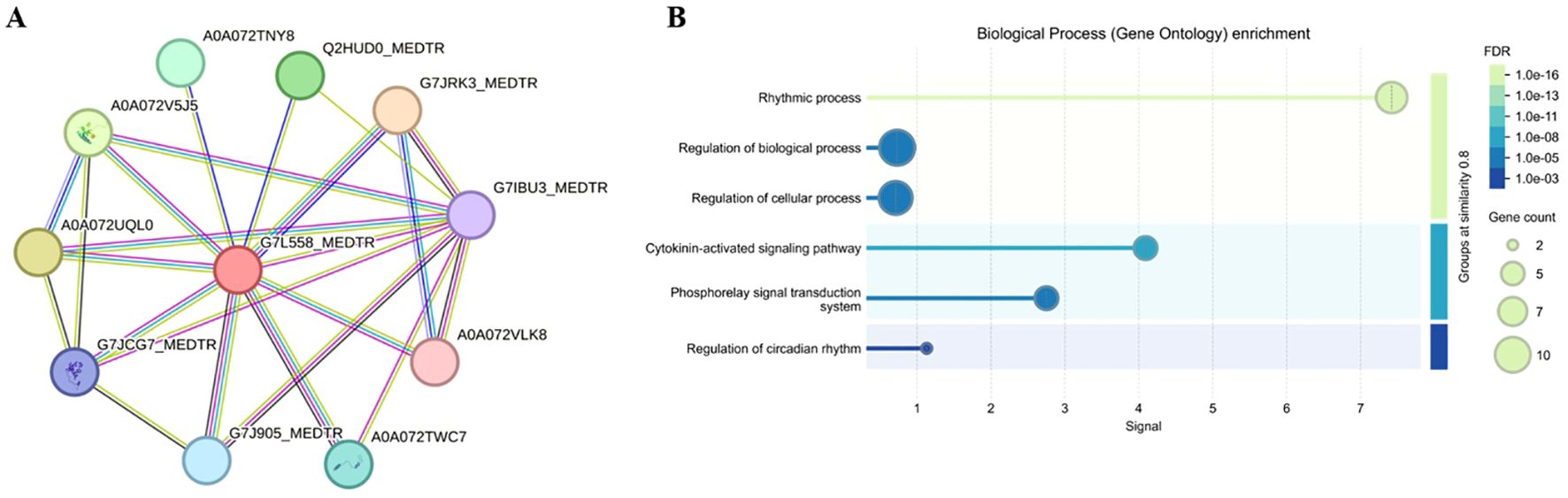
Figure 2. Prediction of MsLHY-interacting proteins using the STRING database. (A) Interacting proteins. (B) Biological process.
3.3 Expression patterns of MsLHY
The relative expression level of MsLHY in different alfalfa tissues was the highest in stems and the lowest in old leaves (Figure 3A). Treatment with different abiotic stresses revealed that MsLHY could be induced by salt, saline–alkaline conditions, drought and low temperature. Compared with that before treatment, after saline–alkaline conditions, drought and salt stress, the relative expression level of MsLHY increased first and then decreased in the above-ground, and the expression level recovered to the level before treatment at 48 h (Figures 3B, D, E). The relative expression of underground parts decreased overall. However, after low-temperature stress, MsLHY was significantly induced in the aboveground parts, and its relative expression level significantly increased within 12 h, reaching 124 times greater than that before treatment at 8 h. The relative expression level of the underground parts reached a maximum at 12 h, i.e., 67 times greater than that before treatment (Figure 3C).
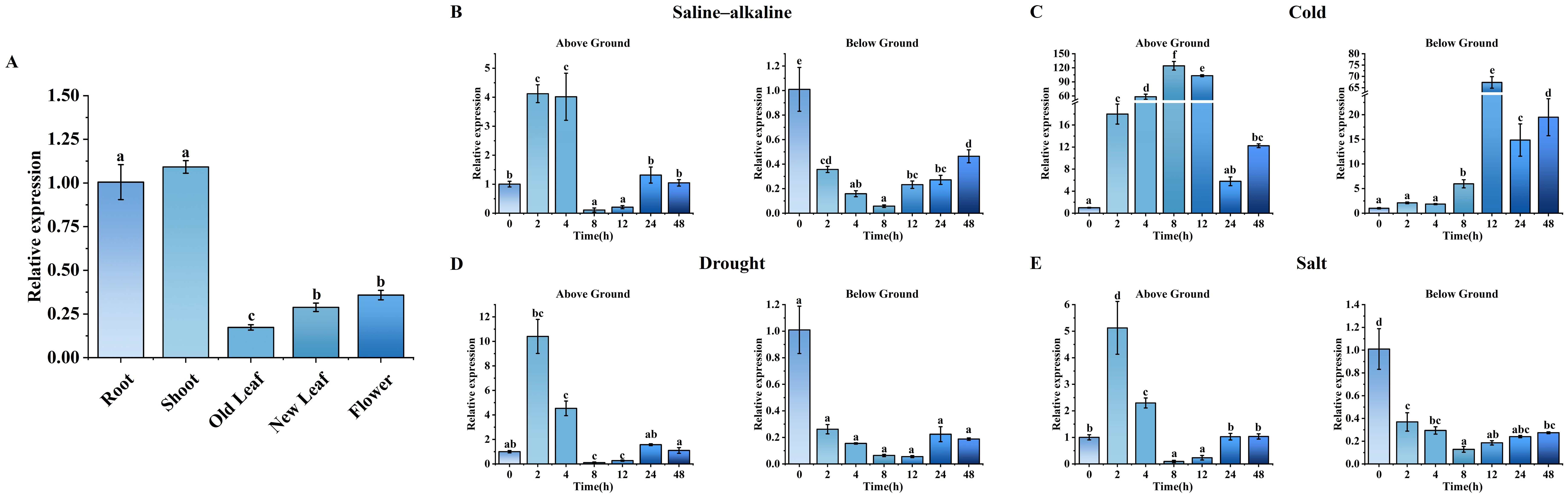
Figure 3. (A) qRT–PCR analysis of MsLHY expression levels in different alfalfa tissues (root, shoot, old leaf, new leaf and flower tissues). (B) Saline–alkaline solution (150 mM NaHCO3). (C) Cold (4°C). (D) Drought (20% PEG-6000). (E) Salt (150 mM NaCl). The error bars represent the means ± SDs (n = 3) from three independent biological replicates. Different letters indicate statistically significant differences (Duncan’s tests, p < 0.05).
3.4 Overexpression of MsLHY increases cold tolerance in transgenic alfalfa
In this study, alfalfa transgenic plants were successfully obtained via the leaf disc method (Supplementary Figure S3). Ten plants positive for overexpression (OE) or RNA interference (RNAi) were screened using Bar test paper and RT–PCR, respectively (Supplementary Figure S4). The expression level of the MsLHY gene was further quantitatively analyzed via qRT–PCR, and three plants were selected on the basis of the expression level for follow-up experiments (Figure 4C). At 4°C, there was no difference in plant phenotype, but at -5°C, the leaves of the RNAi-treated and WT plants wilted, and the OE plants grow normally expect for OE-10, which also showed wilty phenotype, OE-10 showed the lowest over-expression which may not be sufficient to lead to significant phenotype change. Measurement of the relative conductivity of the plants after low-temperature stress revealed that the OE plants presented less ion leakage than the WT and RNAi plants did (Supplementary Figure S5). These results revealed that the overexpression of MsLHY increased the cold tolerance of alfalfa (Figures 4A, B).
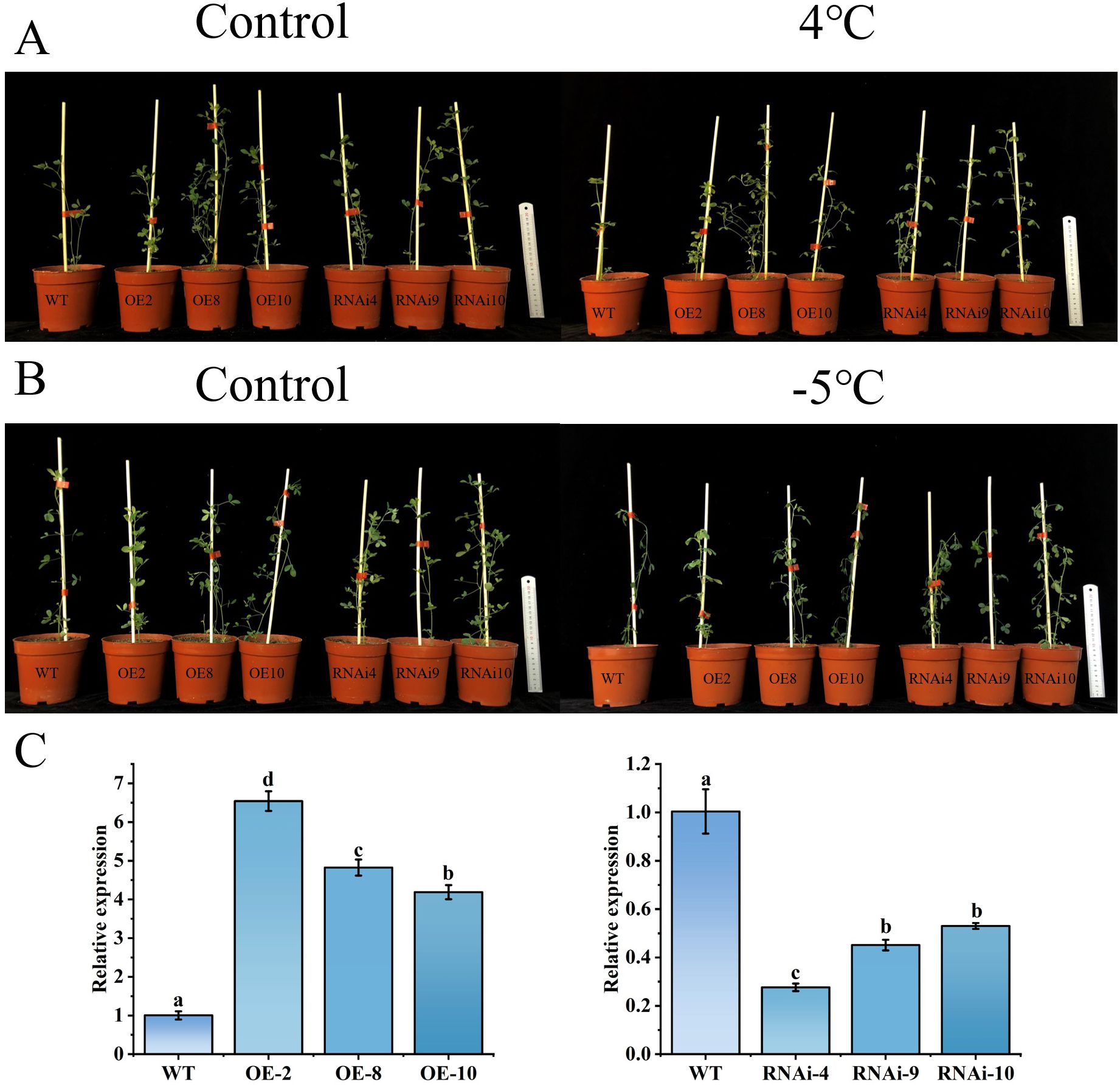
Figure 4. qRT–PCR analysis of the relative expression level of MsLHY in transgenic alfalfa and its phenotype under control conditions and low-temperature stress. (A) Phenotype of transgenic alfalfa treated at 4°C for 7 days. (B) Phenotype of transgenic alfalfa treated at -5°C for 8 hours. (C) Identification of transgenic alfalfa via qRT–PCR. The error bars represent the means ± SDs (n = 3) from three independent biological replicates. Different letters indicate statistically significant differences (Duncan’s tests, p < 0.05).
3.5 Physiological changes in transgenic alfalfa under low-temperature stress
To better verify the function of MsLHY at low temperatures, we measured the physiological indices of the transgenic alfalfa before and after low-temperature stress. The activities of three antioxidant enzymes (SOD, POD, and CAT) in the OE plants were significantly greater than those in the WT and RNAi plants after low-temperature stress (p<0.05) (Figures 5A–C). The CAT activity of the RNAi plants was greater than that of the WT plants after treatment at -5°C and lower than that of the WT plants under other conditions. After low-temperature stress, H2O2 and O2- accumulated in the plants, the content of these molecules in the OE plants was lower than that in the WT plants was, and the content in the RNAi plants was greater than that in the WT plants. In contrast, the content in the OE plants at -5°C was significantly lower than that in the WT plants (p<0.05), indicating that the overexpression of MsLHY reduces the accumulation of reactive oxygen species (ROS) (Figures 5H, I). The MDA content significantly differed between the OE and RNAi plants and the WT plants at -5°C, and the MDA content in the RNAi plants was significantly greater than that in the WT and OE plants (p<0.05) (Figure 5G). Pro plays an important role as an osmotic regulator under low-temperature stress. With the exception of OE-8, the Pro content of the OE plants was significantly greater than that of the WT and RNAi plants (p<0.05) (Figure 5D). Under normal conditions, there was no significant difference in the SP and SS contents of all the plants, but OE and RNAi increased these contents to varying degrees after low-temperature treatment. The SP and SS contents of the OE plants were significantly greater than those of the WT and RNAi plants (p<0.05), and there was no significant difference in the SP and SS contents between the RNAi and WT plants (Figures 5E, F). In conclusion, the overexpression of MsLHY can improve the cold tolerance of alfalfa at the physiological level by increasing the activity of antioxidant enzymes, clearing ROS, and increasing the amount of osmoregulatory substances.
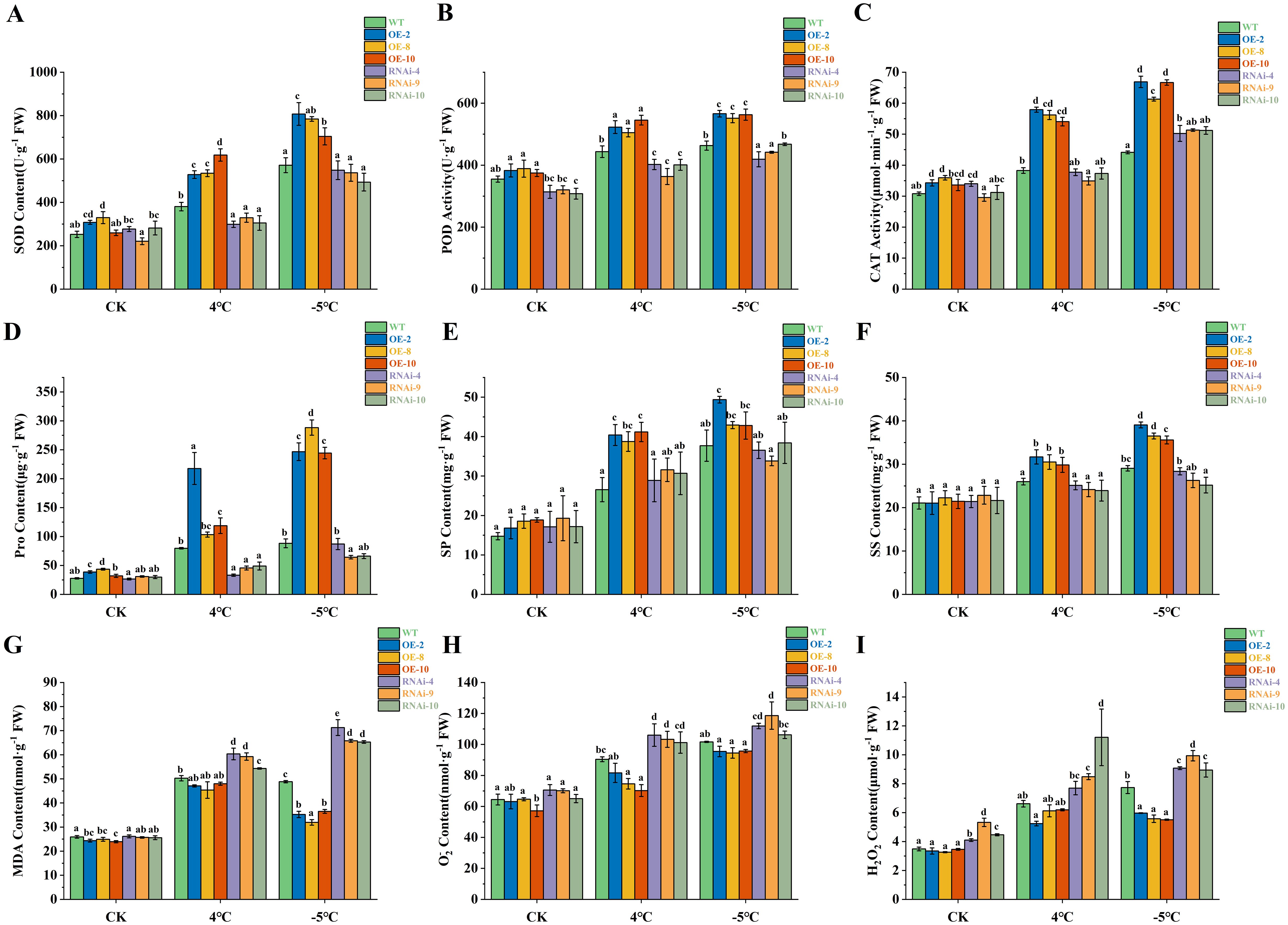
Figure 5. Physiological indices of the control and transgenic plants under 4°C and -5°C stress conditions. The measured indices included the levels of (A) SOD, (B) POD, (C) CAT, (D) Pro, (E) SP, (F) SS, (G) MDA, (H) O2- and (I) H2O2. The error bars represent the means ± SDs (n = 3) from three independent biological replicates. Different letters indicate statistically significant differences (Duncan’s tests, p < 0.05).
3.6 Expression analysis of cold stress-related genes in transgenic alfalfa
The expression patterns of cold stress response genes and antioxidase synthetic genes in alfalfa were analyzed via qRT–PCR at low temperatures. The results revealed that low temperature significantly increased the expression levels of MsICE1, MsCBF1 and MsCOR15A in OE-2 and OE-8 (p<0.05), whereas the expression of only MsCBF1 was significantly increased in OE-10 (p<0.05) (Figures 6A, C, E). Under -5°C stress, the expression levels of MsSOD1 and MsCAT1 were significantly increased in the OE plants (p<0.05) but significantly decreased in the RNAi plants (p<0.05) (Figures 6B, D). Interestingly, MsCAT1 expression in RNAi-4 was significantly greater than that in the WT under -5°C stress. Under normal conditions, the expression of MsCML10 in the RNAi plants was greater than that in the OE plants, but after cold stress, the expression of MsCML10 in the OE plants was significantly greater than that in the RNAi plants (p<0.05) (Figure 6F).
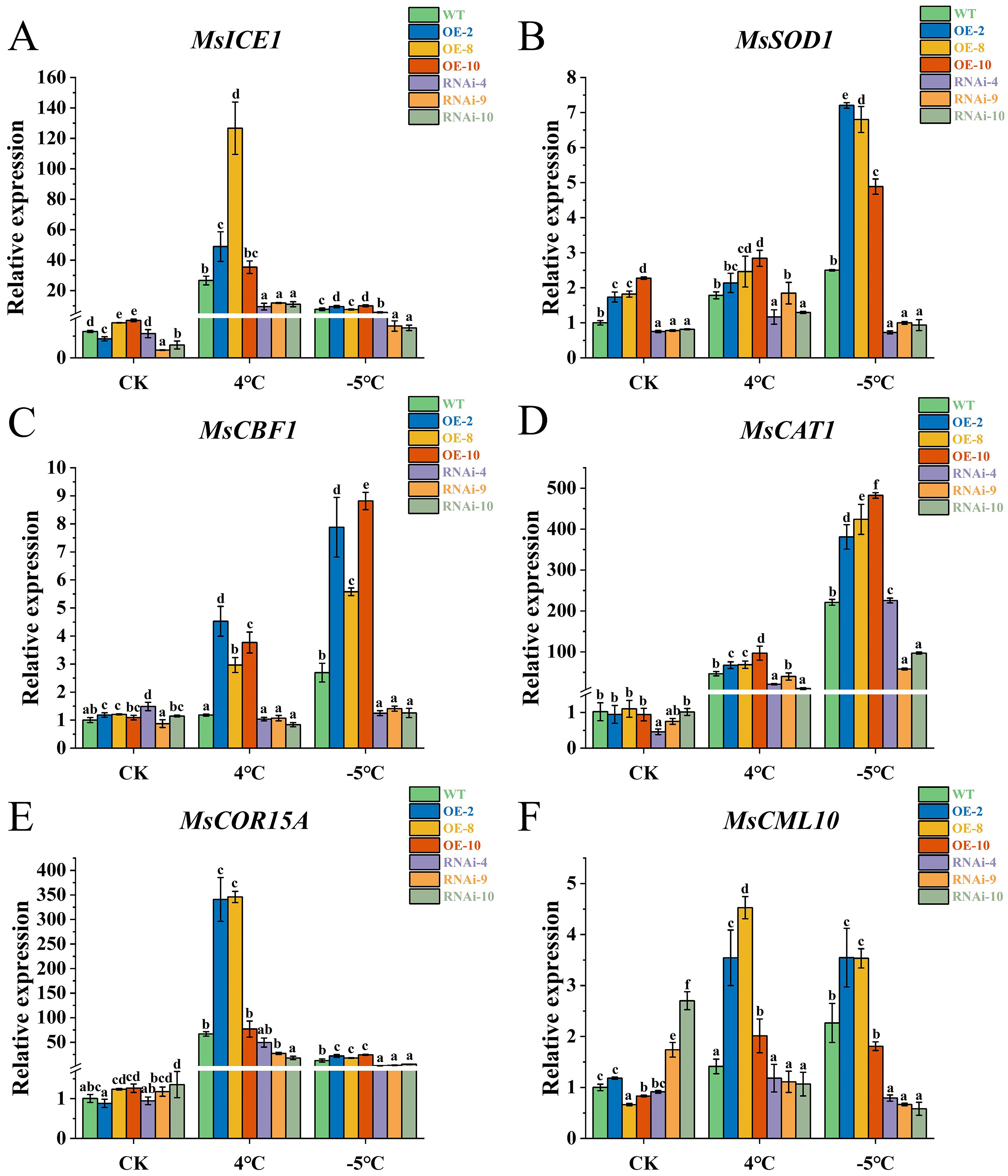
Figure 6. Expression of six related genes in transgenic alfalfa after low-temperature stress. (A) MsICE1, (B) MsSOD1, (C) MsCBF1, (D) MsCAT1, (E) MsCOR15A and (F) MsCML10. The error bars represent the means ± SDs (n = 3) from three independent biological replicates. Different letters indicate statistically significant differences (Duncan’s tests, p < 0.05).
4 Discussion
Temperature is the main environmental factor that determines the geographical distribution of plants and affects their growth and production (Ritonga and Chen, 2020). To cope with cold stress, plants have evolved a series of mechanisms to adapt to cold environments, such as adjusting metabolic processes (Theocharis et al., 2012), changing gene expression patterns (Zhu et al., 2007), synthesizing osmoregulatory substances (Dirk et al., 2020), and epigenetic regulation (Liu et al., 2018; Ding et al., 2020). Like those of the tobacco and Arabidopsis LHY proteins, the N-terminus of MsLHY also has a conserved SANT domain that is a member of the Myb/SANT family (Yon et al., 2012) (Supplementary Figure S1). The expression level of MsLHY was the highest in the stems and the lowest in old leaves, suggesting that MsLHY may play a regulatory role in plant growth and development (Figure 3). Ten interacting proteins enriched in rhythmic processes, cellular processes, biological processes, the phospholipid signal transduction system, and the cytokinin-activated signaling pathway were predicted using the String database (Figure 2). G7JRK3_MEDTR may interact with MsLHY (G7L558_MEDTR). G7JRK3_MEDTR is a PRR, and in A. thaliana, the interaction between TOC1 (also known as PRR1) and CCA1/LHY is reciprocal. CCA1/LHY can bind to the promoter region of TOC1 and inhibit its expression, and TOC1 can indirectly promote the expression of CCA1/LHY by binding the Constans, Constans-like and TOC1 (CCT) domains to the promoters of CCA1/LHY (Alabadi et al., 2001; Mizoguchi et al., 2002; Gendron et al., 2012).
The LHY gene plays an important synergistic role in the abiotic stress process (Wang et al., 2021; Wei et al., 2022) and can induce expression under cold (Nakamichi et al., 2010; Seo et al., 2012), heat (Blair et al., 2019), drought (Wang et al., 2021), salt (Li et al., 2024) and hormone (Zheng et al., 2006) treatments, but the expression of MsLHY in alfalfa under stress has not been studied. In this study, MsLHY was induced by drought, salt and saline–alkaline conditions and was significantly upregulated under cold stress, which was consistent with the expression of OsLHY (Xu et al., 2016) and FmLHY (Cao, 2024) after low-temperature stress. These results suggest that MsLHY can function at low temperatures. Low temperatures can cause damage to plant cell membranes (Aghdam et al., 2019). Through the Agrobacterium-mediated method, we obtained OE and RNAi transgenic alfalfa (Supplementary Figure S3). Under cold stress, the growth of the RNAi plants was inhibited, and the relative conductivity of the RNAi plants was greater than that of the WT plants (Figure 2), indicating that the cold tolerance of alfalfa was reduced due to the inhibition of MsLHY, which was similar to the results of related studies. For example, the cold tolerance of tea plants is reduced by the inhibition of CsLHY (Wu et al., 2024). Studies have shown that when CsLHY is inhibited, the cold tolerance of Camellia sinensis is reduced. SgRVE6 is a transcription factor encoding LHY-CCA1-Like, which can be rapidly upregulated under cold stress, affecting the expression of circadian clock genes and improving the cold tolerance of tobacco (Chen et al., 2020).
Low temperature can lead to the accumulation of ROS in plant cells, and the contents of MDA, H2O2 and O2- reflect the degree of damage caused by low temperature to plants to a certain extent. The results revealed that the increase in the MDA content in cold-resistant sorghum was less than that in cold-sensitive materials (Shi et al., 2015) and that a decrease in the MDA and H2O2 contents improved the cold tolerance of M. sativa (Ye et al., 2024). Plants also remove ROS by increasing the activity of antioxidant enzymes such as SOD and CAT, thereby improving cold resistance (Chen et al., 2014; Wang, 2023). We determined the physiological indices of alfalfa after low-temperature stress and found that the activities of SOD, POD and CAT in OE plants were significantly greater than those in WT plants, whereas the contents of MDA, H2O2 and O2- in RNAi plants were significantly greater than those in WT and OE plants. The enhanced CAT and SOD activities in OE plants were supported by their greater expression levels in plants (Figures 6B, D). These results suggest that the overexpression of MsLHY improves the ability of alfalfa to scavenge ROS and reduces the accumulation of reactive oxygen species. Consistent with findings in Arabidopsis, when the expression of the LHY gene is inhibited, the activity of antioxidant enzymes is reduced, increasing the vulnerability of plants to oxidative damage caused by low-temperature stress (Lai et al., 2012). The accumulation of ROS can also lead to an increase in cell membrane permeability, whereas Pro, SS and SP contents can increase after low-temperature stress to maintain the integrity of the cell membrane, improve the antioxidant capacity (Parvanova et al., 2004; Arminian and Bidgoli, 2019), provide material energy for plant cells (Zhao et al., 2006), and thus increase the cold resistance of plants and reduce the damage caused by low temperature (Luo et al., 2004; Zhu et al., 2020; Sun et al., 2022). In this study, the contents of Pro, SS and SP all increased after low-temperature stress, the contents in OE plants were always significantly greater than those in WT and RNAi plants, and the accumulation at -5°C was greater than that at 4°C. The SS and SP contents of the RNAi plants were the same as those of the WT plants after low-temperature treatment but were still significantly lower than those of the OE plants. These results indicated that low temperature induced the accumulation of osmoregulatory substances to resist cold stress and that the response of plants to freezing injury was greater than that to cold injury.
The ICE-CBF-COR pathway is a key signal transduction pathway involved in the response of plants to low-temperature stress. As the hub of the cold resistance pathway, CBF binds to the CRT/DRE sequence in the promoter of the COR gene under low-temperature conditions and directly promotes transcription in response to low-temperature stress, increasing the ability to resist cold (Tian et al., 2023). Studies have shown that CCA1/LHY can bind to the CBF promoter to play a positive regulatory role (Seo et al., 2012; Nakamichi et al., 2016), and the cold-induced expression of CBF is significantly reduced in Arabidopsis cca1 lhy double mutants (Dong et al., 2011). Inhibition of CsLHY in tea plants decreases the expression of CsCBF1, which in turn decreases the cold tolerance of tea plants (Wu et al., 2024). Under 4°C and -5°C stress, the expression of MsCBF1 in the OE plants was significantly induced and upregulated, whereas the expression in the RNAi plants was significantly lower than that in the WT and OE plants. The expression of MsCOR15A followed the same trend, indicating that MsLHY may mainly regulate downstream MsCOR15A by binding to MsCBF1 to promote cold resistance in alfalfa.
5 Conclusion
We isolated and identified the MsLHY gene in alfalfa. The overexpression of MsLHY increases the activity of antioxidant enzymes and osmoregulatory substances, reduces the accumulation of reactive oxygen species, and improves the cold tolerance of alfalfa with less ion leakage. OE plants under low-temperature stress presented increased expression of cold tolerance genes and antioxidase-encoding genes and increased cold tolerance. In conclusion, MsLHY is an important candidate gene for the development of cold-resistant alfalfa varieties.
Data availability statement
The data presented in the study are included in the article/Supplementary Material.
Author contributions
JL: Conceptualization, Funding acquisition, Resources, Supervision, Writing – review & editing, Data curation, Investigation, Validation, Visualization, Writing – original draft. LC: Data curation, Formal analysis, Methodology, Software, Validation, Visualization, Writing – original draft. MY: Methodology, Validation, Writing – original draft. HZ: Investigation, Methodology, Resources, Writing – original draft. CS: Formal analysis, Software, Writing – original draft. YL: Validation, Visualization, Writing – original draft. KQ: Software, Validation, Writing – original draft. JS: Data curation, Writing – original draft. WH: Project administration, Resources, Writing – review & editing. PZ: Resources, Supervision, Writing – review & editing.
Funding
The author(s) declare that financial support was received for the research and/or publication of this article. This research was funded by the Scientific research operating expenses of Heilongjiang Province (CZKYF2023-1-B008), the Scientific and Technological Innovation Leapfrogging Project of Heilongjiang Academy of Agricultural Sciences (CX22JQ04), the Science and Technology Innovation 2030-Major Project (2022ZD040120403).
Conflict of interest
The authors declare that the research was conducted in the absence of any commercial or financial relationships that could be construed as a potential conflict of interest.
Generative AI statement
The author(s) declare that no Generative AI was used in the creation of this manuscript.
Publisher’s note
All claims expressed in this article are solely those of the authors and do not necessarily represent those of their affiliated organizations, or those of the publisher, the editors and the reviewers. Any product that may be evaluated in this article, or claim that may be made by its manufacturer, is not guaranteed or endorsed by the publisher.
Supplementary material
The Supplementary Material for this article can be found online at: https://www.frontiersin.org/articles/10.3389/fpls.2025.1559988/full#supplementary-material
References
Adams, S., Grundy, J., Veflingstad, S. R., Dyer, N. P., Hannah, M. A., Ott, S., et al. (2018). Circadian control of abscisic acid biosynthesis and signalling pathways revealed by genome-wide analysis of LHY binding targets. New Phytol. 220, 893–907. doi: 10.1111/nph.2018.220.issue-3
Aghdam, M. S., Moradi, M., Razavi, F., Rabiei, V. (2019). Exogenous phenylalanine application promotes chilling tolerance in tomato fruits during cold storage by ensuring supply of NADPH for activation of ROS scavenging systems. Scientia Hortic. 246, 818–825. doi: 10.1016/j.scienta.2018.11.074
Alabadi, D., Oyama, T., Yanovsky, M. J., Harmon, F. G., Mas, P., Kay, S. A. (2001). Reciprocal regulation between TOC1 and LHY/CCA1 within the Arabidopsis circadian clock. Sci. (New York N.Y.) 293, 880–883. doi: 10.1126/science.1061320
Ambroise, V., Legay, S., Guerriero, G., Hausman, J.-F., Cuypers, A., Sergeant, K. (2020). The roots of plant frost hardiness and tolerance. Plant Cell Physiol. 61, 3–20. doi: 10.1093/pcp/pcz196
Annicchiarico, P., Barrett, B., Brummer, E. C., Julier, B., Marshall, A. H. (2015). Achievements and challenges in improving temperate perennial forage legumes. Crit. Rev. Plant Sci. 34, 327–380. doi: 10.1080/07352689.2014.898462
Arminian, A., Bidgoli, R. D. (2019). Simultaneous responses of photosystem II and soluble proteins of rapeseed to cold acclimation. Cell. Mol. Biol. 65, 37–49. doi: 10.14715/cmb/2019.65.2.7
Atamian, H. S., Harmer, S. L. (2016). Circadian regulation of hormone signaling and plant physiology. Plant Mol. Biol. 91, 691–702. doi: 10.1007/s11103-016-0477-4
Blair, E. J., Bonnot, T., Hummel, M., Hay, E., Marzolino, J. M., Quijada, I. A., et al. (2019). Contribution of time of day and the circadian clock to the heat stress responsive transcriptome in Arabidopsis. Sci. Rep. 9, 4814. doi: 10.1038/s41598-019-41234-w
Bremer, A., Kent, B., Hauss, T., Thalhammer, A., Yepuri, N. R., Darwish, T. A., et al. (2017). Intrinsically Disordered Stress Protein COR15A Resides at the Membrane Surface during Dehydration. Biophys. J. 113, 572–579. doi: 10.1016/j.bpj.2017.06.027
Cao, Y. (2024). Study on the F1 selection of Fraxsinus mandshurica Rupr. × Fraxinus americana Linn. and the cold resistance mechanism of FmTCP21 gene (Northeast Forestry University).
Chen, S., Huang, H. A., Chen, J. H., Fu, C. C., Zhan, P. L., Ke, S. W., et al. (2020). SgRVE6, a LHY-CCA1-like transcription factor from fine-stem stylo, upregulates NB-LRR gene expression and enhances cold tolerance in tobacco. Front. Plant Sci. 11. doi: 10.3389/fpls.2020.01276
Chen, S., Sun, J., Ma, W., Wang, J., Zhao, X., Hu, R. (2022). Regulation mechanism of low temperature stress on plants: research progress. Chin. Agric. Sci. Bull. 38, 51–61. doi: 10.11924/j.issn.1000-6850.cabs-2021-0656
Chen, Y., Jiang, J. F., Chang, Q. S., Gu, C. S., Song, A. P., Chen, S. M., et al. (2014). Cold acclimation induces freezing tolerance via antioxidative enzymes, proline metabolism and gene expression changes in two chrysanthemum species. Mol. Biol. Rep. 41, 815–822. doi: 10.1007/s11033-013-2921-8
Ding, Y., Shi, Y., Yang, S. (2019). Advances and challenges in uncovering cold tolerance regulatory mechanisms in plants. New Phytol. 222, 1690–1704. doi: 10.1111/nph.2019.222.issue-4
Ding, Y., Shi, Y., Yang, S. (2020). Molecular regulation of plant responses to environmental temperatures. Mol. Plant 13, 544–564. doi: 10.1016/j.molp.2020.02.004
Dirk, L. M. A., Abdel, C. G., Ahmad, I., Silva Neta, I. C., Pereira, C. C., Carlos Bezerra Pereira, F. E., et al. (2020). Late embryogenesis abundant protein-client protein interactions. Plants-Basel 9, 814. doi: 10.3390/plants9070814
Dong, M. A., Farre, E. M., Thomashow, M. F. (2011). CIRCADIAN CLOCK-ASSOCIATED 1 and LATE ELONGATED HYPOCOTYL regulate expression of the C-REPEAT BINDING FACTOR (CBF) pathway in Arabidopsis. Proc. Natl. Acad. Sci. United States America 108, 7241–7246. doi: 10.1073/pnas.1103741108
Farre, E. M., Liu, T. (2013). The PRR family of transcriptional regulators reflects the complexity and evolution of plant circadian clocks. Curr. Opin. Plant Biol. 16, 621–629. doi: 10.1016/j.pbi.2013.06.015
Gendron, J. M., Pruneda-Paz, J. L., Doherty, C. J., Gross, A. M., Kang, S. E., Kay, S. A. (2012). Arabidopsis circadian clock protein, TOC1, is a DNA-binding transcription factor. Proc. Natl. Acad. Sci. United States America 109, 3167–3172. doi: 10.1073/pnas.1200355109
Grabelnych, O. I., Borovik, O. A., Tauson, E. L., Pobezhimova, T. P., Katyshev, A. I., Pavlovskaya, N. S., et al. (2014). Mitochondrial energy-dissipating systems (alternative oxidase, uncoupling proteins, and external NADH dehydrogenase) are involved in development of frost-resistance of winter wheat seedlings. Biochemistry-Moscow 79, 506–519. doi: 10.1134/S0006297914060030
Guo, J., Ren, Y., Tang, Z., Shi, W., Zhou, M. (2019). Characterization and expression profiling of the ICE-CBF-COR genes in wheat. Peerj 7, e8190. doi: 10.7717/peerj.8190
Harmer, S. L., Kay, S. A. (2005). Positive and negative factors confer phase-specific circadian regulation of transcription in Arabidopsis. Plant Cell 17, 1926–1940. doi: 10.1105/tpc.105.033035
Hung, F.-Y., Chen, F.-F., Li, C., Chen, C., Chen, J.-H., Cui, Y., et al. (2019). The LDL1/2-HDA6 histone modification complex interacts with TOC1 and regulates the core circadian clock components in arabidopsis. Front. Plant Sci. 10. doi: 10.3389/fpls.2019.00233
Ke, L., Gu, H., Qu, L. (1998). Plant molecular biology: an experimental manual (Heidelberg: Springer).
Kyung, J., Jeon, M., Jeong, G., Shin, Y., Seo, E., Yu, J., et al. (2022). The two clock proteins CCA1 and LHY activate VIN3 transcription during vernalization through the vernalization-responsive cis-element. Plant Cell 34, 1020–1037. doi: 10.1093/plcell/koab304
Lai, A. G., Doherty, C. J., Mueller-Roeber, B., Kay, S. A., Schippers, J. H. M., Dijkwel, P. P. (2012). CIRCADIAN CLOCK-ASSOCIATED 1 regulates ROS homeostasis and oxidative stress responses. Proc. Natl. Acad. Sci. United States America 109, 17129–17134. doi: 10.1073/pnas.1209148109
Lee, C. M., Thomashow, M. F. (2012). Photoperiodic regulation of the C-repeat binding factor (CBF) cold acclimation pathway and freezing tolerance in Arabidopsis thaliana. Proc. Natl. Acad. Sci. United States America 109, 15054–15059. doi: 10.1073/pnas.1211295109
Li, C., He, Y.-Q., Yu, J., Kong, J.-R., Ruan, C.-C., Yang, Z.-K., et al. (2024). The rice LATE ELONGATED HYPOCOTYL enhances salt tolerance by regulating Na+/K+ homeostasis and ABA signalling. Plant Cell Environ. 47, 1625–1639. doi: 10.1111/pce.14835
Liu, X., Li, G., Cui, G. (2006). Study on the relationship between early growth of alfalfa and its cold resistance. Modern Anim. Husbandry Sci. Technol. 12, 52–53. doi: 10.11686/cyxb2021299
Liu, J., Shi, Y., Yang, S. (2018). Insights into the regulation of C-repeat binding factors in plant cold signaling. J. Integr. Plant Biol. 60, 780–795. doi: 10.1111/jipb.12657
Liu, M., Wu, Y., Niu, Z., Sun, Y., Yue, X. (2024). Modeling and analysis of photoperiodic flowering in arabidopsis thaliana. Hans J. Comput. Biol. 13, 23. doi: 10.12677/hjcb.2023.133003
Livak, K. J., Schmittgen, T. D. (2001). Analysis of relative gene expression data using real-time quantitative PCR and the 2(-Delta Delta C(T)) Method. Methods (San Diego Calif.) 25, 402–408. doi: 10.1006/meth.2001.1262
Luo, X., Feng, C., Li, H., Sha, W. (2004). Study on Changes of SOD and Proline Activity during Low temperature Stress on Medicago sativa L.cv.Zhaodong. Chin. J. Grassland 04, 79–81. doi: 10.3321/j.issn:1673-5021.2004.04.015
Mickelbart, M. V., Hasegawa, P. M., Bailey-Serres, J. (2015). Genetic mechanisms of abiotic stress tolerance that translate to crop yield stability. Nat. Rev. Genet. 16, 237–251. doi: 10.1038/nrg3901
Mi-Jeong, P., Young-Ju, K., Kyung-Eun, G., Chung-Mo, P. (2016). LATE ELONGATED HYPOCOTYL regulates photoperiodic flowering via the circadian clock in Arabidopsis. BMC Plant Biol. 16, 114. doi: 10.1186/s12870-016-0810-8
Mizoguchi, T., Wheatley, K., Hanzawa, Y., Wright, L., Mizoguchi, M., Song, H. R., et al. (2002). LHY and CCA1 are partially redundant genes required to maintain circadian rhythms in Arabidopsis. Dev. Cell 2, 629–641. doi: 10.1016/S1534-5807(02)00170-3
Murata, N., Takahashi, S., Nishiyama, Y., Allakhverdiev, S. I. (2007). Photoinhibition of photosystem II under environmental stress. Biochim. Et Biophys. Acta-Bioenergetics 1767, 414–421. doi: 10.1016/j.bbabio.2006.11.019
Nakamichi, N., Kiba, T., Henriques, R., Mizuno, T., Chua, N.-H., Sakakibara, H. (2010). PSEUDO-RESPONSE REGULATORS 9, 7, and 5 are transcriptional repressors in the arabidopsis circadian clock. Plant Cell 22, 594–605. doi: 10.1105/tpc.109.072892
Nakamichi, N., Takao, S., Kudo, T., Kiba, T., Wang, Y., Kinoshita, T., et al. (2016). Improvement of arabidopsis biomass and cold, drought and salinity stress tolerance by modified circadian clock-associated PSEUDO-RESPONSE REGULATORs. Plant Cell Physiol. 57, 1085–1097. doi: 10.1093/pcp/pcw057
Parvanova, D., Ivanov, S., Konstantinova, T., Karanov, E., Atanassov, A., Tsvetkov, T., et al. (2004). Transgenic tobacco plants accumulating osmolytes show reduced oxidative damage under freezing stress. Plant Physiol. Biochem. 42, 57–63. doi: 10.1016/j.plaphy.2003.10.007
Ritonga, F. N., Chen, S. (2020). Physiological and molecular mechanism involved in cold stress tolerance in plants. Plants-Basel 9, 560. doi: 10.3390/plants9050560
Seo, P. J., Park, M.-J., Lim, M.-H., Kim, S.-G., Lee, M., Baldwin, I. T., et al. (2012). A self-regulatory circuit of CIRCADIAN CLOCK-ASSOCIATED1 underlies the circadian clock regulation of temperature responses in arabidopsis. Plant Cell 24, 2427–2442. doi: 10.1105/tpc.112.098723
Shi, Y. T., Ding, Y. L., Yang, S. H. (2018). Molecular reculation of CBF sicnalinc in colc acclimation. Trends Plant Sci. 23, 623–637. doi: 10.1016/j.tplants.2018.04.002
Shi, H., Zhang, H., Yang, B., Zhang, G. (2015). Effects of low temperature stress on the content of MDA, SOD and POD activity in sorghum seedlings. Chin. Agric. Sci. Bull. 31, 74–79. doi: 10.11924/j.issn.1000-6850.casb14120134
Shu, Y., Li, W., Zhao, J., Zhang, S., Xu, H., Liu, Y., et al. (2017). Transcriptome sequencing analysis of alfalfa reveals CBF genes potentially playing important roles in response to freezing stress. Genet. Mol. Biol. 40, 824–833. doi: 10.1590/1678-4685-gmb-2017-0053
Song, L. L., Ding, W., Zhao, M. G., Sun, B. T., Zhang, L. X. (2006). Nitric oxide protects against oxidative stress under heat stress in the calluses from two ecotypes of reed. Plant Sci. 171, 449–458. doi: 10.1016/j.plantsci.2006.05.002
Su, C., Wang, Y., Yu, Y., He, Y., Wang, L. (2021). Coordinative regulation of plants growth and development by light and circadian clock. aBIOTECH 2, 176–189. doi: 10.1007/s42994-021-00041-6
Sun, M., Zhang, Y., Xia, Q., Wang, X., Zhang, Q., Liu, T., et al. (2022). Effects of potash fertilizer types and rates on alfalfa root crown saccharides content and cold resistance under low temperature stress. Agric. Res. Arid Areas 40, 62–70. doi: 10.7606/j.issn.1000-7601.2022.05.07
Theocharis, A., Clement, C., Barka, E. A. (2012). Physiological and molecular changes in plants grown at low temperatures. Planta 235, 1091–1105. doi: 10.1007/s00425-012-1641-y
Thomashow, M. F. (1999). PLANT COLD ACCLIMATION: freezing tolerance genes and regulatory mechanisms. Annu. Rev. Plant Physiol. Plant Mol. Biol. 50, 571–599. doi: 10.1146/annurev.arplant.50.1.571
Tian, Y., Jiang, M., Zhang, Q. (2023). Progress on cold resistance genes and ICE-CBF-COR signaling in plants. Agric. Eng. 13, 131–137. doi: 10.19998/j.cnki.2095-1795.2023.05.024
Wang, M. (2023). Comparative Study on Physiological Characteristics and Transcriptome of Different Alfalfa Varieties in Response to Low Temperature (Master, Northeast Agricultural University).
Wang, K., Bu, T., Cheng, Q., Dong, L., Su, T., Chen, Z., et al. (2020). Two homologous LHY pairs negatively control soybean drought tolerance by repressing the abscisic acid responses. New Phytol. 229, 2660–2675. doi: 10.1111/nph.v229.5
Wang, K., Bu, T., Cheng, Q., Dong, L., Su, T., Chen, Z., et al. (2021). Two homologous LHY pairs negatively control soybean drought tolerance by repressing the abscisic acid responses. New Phytol. 229, 2660–2675. doi: 10.1111/nph.v229.5
Wang, D.-Z., Jin, Y.-N., Ding, X.-H., Wang, W.-J., Zhai, S.-S., Bai, L.-P., et al. (2017). Gene regulation and signal transduction in the ICE-CBF-COR signaling pathway during cold stress in plants. Biochemistry-Moscow 82, 1103–1117. doi: 10.1134/S0006297917100030
Wang, Q., Wu, J., Di, G., Zhao, Q., Gao, C., Zhang, D., et al. (2024). Identification of Cold Tolerance Transcriptional Regulatory Genes in Seedlings of Medicago sativa L. and Medicago falcata L. Int. J. Mol. Sci. 25, 10345. doi: 10.3390/ijms251910345
Wei, H., Xu, H., Su, C., Wang, X., Wang, L. (2022). Rice CIRCADIAN CLOCK ASSOCIATED 1 transcriptionally regulates ABA signaling to confer multiple abiotic stress tolerance. Plant Physiol. 190, 1057–1073. doi: 10.1093/plphys/kiac196
Wu, Y., Di, T., Wu, Z., Peng, J., Wang, J., Zhang, K., et al. (2024). CsLHY positively regulates cold tolerance by activating CsSWEET17 in tea plants. Plant Physiol. Biochem. 207, 108341. doi: 10.1016/j.plaphy.2024.108341
Xu, J., Jiang, H., Lin, H., Huang, M., Fu, Q., Zeng, D., et al. (2016). EARLY SENESCENCE 1 participates in the expression regulation of circadian clock genes and response to stress in rice. Chin. Bull. Bot. 51, 743–756. doi: 10.11983/CBB16019
Xu, X., Yuan, L., Xie, Q. (2022). The circadian clock ticks in plant stress responses. Stress Biol. 2, 15–15. doi: 10.1007/s44154-022-00040-7
Ye, Q. Y., Zheng, L. H., Liu, P., Liu, Q. W., Ji, T., Liu, J. L., et al. (2024). The S-acylation cycle of transcription factor MtNAC80 influences cold stress responses in Medicago truncatula. Plant Cell 36, 2629–2651. doi: 10.1093/plcell/koae103
Yon, F., Seo, P.-J., Ryu, J. Y., Park, C.-M., Baldwin, I. T., Kim, S.-G. (2012). Identification and characterization of circadian clock genes in a native tobacco, Nicotiana attenuata. BMC Plant Biol. 12, 172. doi: 10.1186/1471-2229-12-172
Yu, S., Wu, J., Sun, Y., Zhu, H., Sun, Q., Zhao, P., et al. (2022). A calmodulin-like protein (CML10) interacts with cytosolic enzymes GSTU8 and FBA6 to regulate cold tolerance. Plant Physiol. 190, 1321–1333. doi: 10.1093/plphys/kiac311
Zhang, P. (2014). ROOT NODULES CONFER SALTTOLERANCE UPON ALFALFA (MEDICAGO SATIVA L.) PLANTS, PROTEOME ANALYSIS (Northwest A&F University).
Zhao, J., Li, X., Li, H. (2006). Research on the role of the soluble sugar in the regulation of physiological metabolism in higher plant. J. Anhui Agric. Sci. 24, 6423–6425 + 6427. doi: 10.3969/j.issn.0517-6611.2006.24.008
Zheng, B., Deng, Y., Mu, J., Ji, Z., Xiang, T., Niu, Q.-W., et al. (2006). Cytokinin affects circadian-clock oscillation in a phytochrome B- and Arabidopsis response regulator 4-dependent manner. Physiologia Plantarum 127, 277–292. doi: 10.1111/j.1399-3054.2006.00660.x
Zhu, J., Dong, C.-H., Zhu, J.-K. (2007). Interplay between cold-responsive gene regulation, metabolism and RNA processing during plant cold acclimation. Curr. Opin. Plant Biol. 10, 290–295. doi: 10.1016/j.pbi.2007.04.010
Keywords: Medicago sativa L., late-elongated hypocotyl, low temperature, interacting protein, transgenic alfalfa
Citation: Li J, Chai L, Yang M, Zhang H, Shang C, Liu Y, Qian K, Sun J, Han W and Zhang P (2025) MsLHY is an active regulator of cold resistance in alfalfa (Medicago sativa L.). Front. Plant Sci. 16:1559988. doi: 10.3389/fpls.2025.1559988
Received: 13 January 2025; Accepted: 07 March 2025;
Published: 01 May 2025.
Edited by:
Linkai Huang, Sichuan Agricultural University, ChinaReviewed by:
Yajun Wu, South Dakota State University, United StatesTianzuo Wang, Chinese Academy of Sciences (CAS), China
Copyright © 2025 Li, Chai, Yang, Zhang, Shang, Liu, Qian, Sun, Han and Zhang. This is an open-access article distributed under the terms of the Creative Commons Attribution License (CC BY). The use, distribution or reproduction in other forums is permitted, provided the original author(s) and the copyright owner(s) are credited and that the original publication in this journal is cited, in accordance with accepted academic practice. No use, distribution or reproduction is permitted which does not comply with these terms.
*Correspondence: Weibo Han, MTM5MzYyNTM5NjBAMTYzLmNvbQ==; Pan Zhang, emhhbmdwYW5AbmVhdS5lZHUuY24=
†These authors have contributed equally to this work and share first authorship