- 1Department of Agronomy and Plant Genetics, University of Minnesota, St Paul, MN, USA
- 2Department of Plant, Soil and Microbial Sciences, Michigan State University, East Lansing, MI, USA
- 3Department of Natural Resources and the Environment, University of New Hampshire, Durham, NH, USA
- 4Department of Natural Resources and Environmental Sciences, University of Illinois at Urbana–Champaign, Urbana, IL, USA
- 5Global Change and Photosynthesis Research Unit, United States Department of Agriculture – Agricultural Research Service, Urbana, IL, USA
- 6Department of Plant Science, The Pennsylvania State University, University Park, PA, USA
- 7Department of Biology, Brigham Young University, Provo, UT, USA
- 8Soil and Water Management Unit, United States Department of Agriculture – Agricultural Research Service, St Paul, MN, USA
There is increasing global demand for food, bioenergy feedstocks and a wide variety of bio-based products. In response, agriculture has advanced production, but is increasingly depleting soil regulating and supporting ecosystem services. New production systems have emerged, such as no-tillage, that can enhance soil services but may limit yields. Moving forward, agricultural systems must reduce trade-offs between production and soil services. Soil functional zone management (SFZM) is a novel strategy for developing sustainable production systems that attempts to integrate the benefits of conventional, intensive agriculture, and no-tillage. SFZM creates distinct functional zones within crop row and inter-row spaces. By incorporating decimeter-scale spatial and temporal heterogeneity, SFZM attempts to foster greater soil biodiversity and integrate complementary soil processes at the sub-field level. Such integration maximizes soil services by creating zones of ‘active turnover’, optimized for crop growth and yield (provisioning services); and adjacent zones of ‘soil building’, that promote soil structure development, carbon storage, and moisture regulation (regulating and supporting services). These zones allow SFZM to secure existing agricultural productivity while avoiding or minimizing trade-offs with soil ecosystem services. Moreover, the specific properties of SFZM may enable sustainable increases in provisioning services via temporal intensification (expanding the portion of the year during which harvestable crops are grown). We present a conceptual model of ‘virtuous cycles’, illustrating how increases in crop yields within SFZM systems could create self-reinforcing feedback processes with desirable effects, including mitigation of trade-offs between yield maximization and soil ecosystem services. Through the creation of functionally distinct but interacting zones, SFZM may provide a vehicle for optimizing the delivery of multiple goods and services in agricultural systems, allowing sustainable temporal intensification while protecting and enhancing soil functioning.
Introduction
Intensification of agriculture has been vital for increasing global food supply and alleviating hunger for millions of people (Godfray et al., 2010). In addition, intensification is key to meeting growing demand for bioenergy feedstocks and a wide variety of bio-based products (Jordan et al., 2007; McCormick and Kautto, 2013). However, agricultural intensification has also resulted in damage to the environment. In particular, soils in many regions of the world have been degraded by intensive agricultural practices (Mäder et al., 2002; Tilman et al., 2002; Heenan et al., 2004), and this has led to increased societal demand for more sustainable agricultural production systems (Foley et al., 2011; Kremen and Miles, 2012). In response, new management strategies have emerged, including soil-focused approaches such as no-tillage, which aim to improve soil regulating and supporting ecosystem services by reducing soil disturbance (Hobbs et al., 2008; Baveye et al., 2011; Palm et al., 2014). However, no-tillage often results in reduced yields (Giller et al., 2009; Pittelkow et al., 2015), highlighting trade-offs between soil and provisioning services. Such trade-offs are highly problematic, given that global demand for food and other agricultural products is expected to rise considerably by 2050 (Godfray et al., 2010; Tilman et al., 2011). Furthermore, to limit the need to convert additional lands to agriculture (i.e., extensification), the world’s existing crop production systems must become more productive (Foley et al., 2011; Bommarco et al., 2013; Godfray and Garnett, 2014).
One option for securing the productivity of existing agricultural land while also enhancing delivery of soil ecosystem services is by integrating the high productivity of intensive field crop production systems (including intensive tillage) with the improvements in soil quality associated with stringent limitations on tillage. Herein, we present evidence that a novel approach to management of field crop agroecosystems – soil functional zone management (SFZM) – can promote such integration. As detailed below, SFZM entails the creation and management of distinct yet complementary soil functional zones that have potential to reduce trade-offs between short-term productivity and soil quality.
We believe SFZM to be a previously unrecognized strategy for expanding the range of ecosystem service production from field crop agroecosystems. Several forms of SFZM (e.g., ridge tillage and strip tillage) have been studied extensively in terms of their effects on a range of crop and soil attributes. Here, we expand upon this level of analysis and understanding through a broad exploration of ecosystem service production and underlying agroecological processes in SFZM, drawing on a wide range of evidence and identifying critical knowledge gaps in understanding of SFZM. In our analysis, we focus first on supporting and regulating services, and then examine the potential of SFZM to increase productivity of agricultural systems (i.e., enhance provisioning services). In particular, we consider the role of SFZM in supporting temporal intensification, which aims to enhance provisioning services by expanding the annual time period in which harvestable crops are grown. We consider the potential dynamics of agroecosystems under SFZM, and the role of these dynamics in improving the sustainability of temporal intensification. We focus on the dynamic implications of ‘virtuous cycles’ (self-reinforcing feedback processes with desirable effects) that may occur in SFZM. Such feedback processes may serve to reduce trade-offs between provisioning, supporting, and regulating services in temporal intensification.
Soil Functional Zone Management
Soil functional zone management is a novel concept of field crop agroecosystem management that seeks to create distinct, yet functionally complementary soil zones through non-uniform management of tillage and crop residues. These zones can be tailored for a variety of different functions or ecosystem services and can be permanent or change locations between seasons. At its most basic, SFZM involves a zone of ‘active turnover’, managed to optimize conditions for seed germination and crop growth; and an adjacent ‘soil building’ zone, which is managed to protect soil organic matter (SOM), enhance soil water holding capacity and provide habitats for soil organisms. At present, the two most widely practiced implementations of SFZM are ridge tillage and strip tillage (Figure 1). While SFZM does not necessarily involve novel management practices (e.g., ridge tillage has been practiced since the 1980s), it provides a novel framework for enhancing ecosystem service production in field crop agroecosystems.
Soil functional zone management differs markedly from conventional and no-tillage practices, which can both be characterized as non-zonal, or uniform. For example, in a chisel plow system, topsoil and crop residues are uniformly mixed, creating a relatively homogenous soil environment across a tilled field (Mannering and Fenster, 1983). In no-tillage, the soil is left undisturbed and crop residues are retained, providing uniform residue cover on the soil surface (Mannering and Fenster, 1983; Hobbs et al., 2008; Figure 1). Despite advances in precision agricultural application of fertilizer and agrochemicals, tillage is still predominantly applied homogeneously (Lal, 2015).
Through creation and management of differentiated soil zones (Figure 1), SFZM creates spatial heterogeneity over small (<1 m) spatial scales. Relative to non-zonal tillage, such enhancement of within-field heterogeneity across space and time serves to enhance the range of soil physical conditions and functional biodiversity within a row-crop agroecosystem. Increasing heterogeneity can enhance biodiversity by providing habitat and other key resources to a wider range of organisms. This expansion of resource diversity in space and time can support effective resource partitioning and increased diversity of microhabitats, allowing coexistence of soil organisms and increased functional biodiversity (Ettema and Wardle, 2002; Kremen and Miles, 2012). In turn, increased functional biodiversity can support provisioning services while simultaneously conserving or enhancing a range of soil services, including organic matter decomposition and nutrient turnover, soil carbon storage, and pathogen suppression (Coleman et al., 2004; Birkhofer et al., 2008; van der Heijden et al., 2008; Suzuki et al., 2013).
Conventional soil management is typically characterized by frequent and intense disturbance (e.g., tillage and agrochemicals) combined with low plant resource diversity (e.g., monocultures and minimal crop residue). These factors lead to reduced abundances and diversity of soil organisms in conventional systems compared with no-tillage and other systems with reduced tillage and more diverse crop rotations (Wardle, 1995; Kabir, 2005; Culman et al., 2010; Postma-Blaauw et al., 2010). Moreover, these factors selectively alter soil biotic communities, leading to dominance by r-selected organisms (organisms adapted for rapid reproduction and dispersal; Pianka, 1970; Verbruggen and Kiers, 2010). For example, larger-bodied soil organisms are reduced in abundance relative to smaller-bodied organisms, leading to reductions in faunal and fungal biomass, and shifts toward bacterial dominance (de Vries et al., 2006; Postma-Blaauw et al., 2010). The adoption of no-tillage management has been demonstrated to improve the abundance and diversity of soil communities, such that they more closely resemble undisturbed grasslands (Postma-Blaauw et al., 2012; Säle et al., 2015). SFZM entails limited and targeted disturbance across both space and time, and maintenance of crop residues, thereby providing undisturbed or minimally disturbed soil refugia (Figure 1). We hypothesize that these refugia can support faunal and fungal diversity in a similar way to no-tillage, and provide a base from which slow-growing organisms with longer generation times (K-selected organisms) might be able to recolonize disturbed areas. In essence, we propose that SFZM, by expanding both habitat and resources relative to conventional soil management, can enhance both provisioning and soil regulating and supporting services by enhancing soil biodiversity.
SFZM and Soil Ecosystem Services
Securing high levels of agricultural production while simultaneously improving regulating and supporting soil ecosystem services requires management strategies that expand the range of service production (Foley et al., 2011; Bommarco et al., 2013). As outlined above, by providing spatial and temporal heterogeneity in terms of tillage and crop residue distribution, we hypothesize that SFZM is one such strategy. In the following sections, we present and examine evidence that SFZM can, in fact, enhance soil ecosystem service delivery.
Supporting Soil Services
Services Produced by Soil Biota
The creation of undisturbed refugia for soil microbiota, particularly filamentous fungi, through targeted disturbance is one pathway by which SFZM may increase the supply of supporting soil services. Such refugia should impact carbon (C), nitrogen (N), and phosphorus (P) cycling to the benefit of above-ground productivity. Nutrient cycling among organic and inorganic pools is driven by microbial turnover, with fungi generally thought to be more effective at storing C and N in organic matter than bacteria (Six et al., 2006), while the higher turnover rate of bacteria promotes gross mineralization and plant nutrient uptake (Schimel and Bennett, 2004). Filamentous, saprophytic fungi are also the dominant decomposers of recalcitrant plant litter, producing more degradative enzymes than bacteria (Treseder and Lennon, 2015). Arbuscular mycorrhizal fungi (AMF), meanwhile, are well-known to dominate plant P nutrition, and their central role in C and N cycling is increasingly recognized (Hodge and Storer, 2015). Thus, a combination of bacteria- and fungi-rich communities is desirable for efficient nutrient cycling.
Soil communities under conventional tillage generally have altered structural, morphological, and functional profiles compared to communities under no-tillage. Overall, tillage lowers microbial biomass, enzyme activities, and nutrient cycling rates (Kladivko, 2001; Balota et al., 2014). While tillage does not necessarily alter fungal:bacterial ratios directly, as bacterial biomass also tends to decrease with tillage (Strickland and Rousk, 2010), lower levels of soil moisture under conventional tillage do reduce fungal:bacterial ratios (Frey et al., 1999). As well, tillage reduces AMF community diversity, creating lower diversity subsets of no-tillage communities (Verbruggen et al., 2012). Those AMF that remain are r-selected, producing more reproductive spores and fewer soil-exploring hyphae than K-selected AMF (Verbruggen and Kiers, 2010). The r-selected AMF recover quickly from disturbance but are less efficient at delivering resources to crops (Powell et al., 2009; Verbruggen and Kiers, 2010).
Under SFZM, both disturbed and undisturbed regions are directly adjacent to each other (Figure 1). The disturbed region exposes labile organic matter and aerates the soil, providing excellent conditions for nutrient turnover immediately after disturbance (Martens, 2001), while the undisturbed region creates a refuge for slower-growing, more sensitive filamentous fungi and hyphae-intensive AMF. From this refuge, these organisms can quickly re-colonize the mixed and aerated disturbed region. The ‘refuge and recolonization’ process may enhance organic matter production and nutrient cycling. Slow-growing K-selected fungi contribute to long-term organic matter pools through necromass production and through the formation of protective soil aggregates (Six et al., 2006; Crowther et al., 2015; Ludwig et al., 2015). As primary decomposers of crop residues, they also have unique ability to access N-rich soil and C-rich crop residues simultaneously, transporting C from residue to soil, and N from soil to residue (Hendrix et al., 1986; Frey et al., 2000, 2003). Tillage disrupts the hyphal networks of these fungi, thereby limiting the production of these services. However, disturbance does enhance residue-soil contact to speed colonization by decomposers. Therefore, the creation of two functionally distinct, adjacent zones under SFZM – an undisturbed fungal refuge and an area where residue is mixed well with soil – should facilitate decomposition of crop residue and the formation of organic matter.
Such refugia may explain enhanced P delivery to maize (Zea mays L.) by AMF in SFZM systems (McGonigle and Miller, 1993). P-limitation is a common problem for cereal production in many temperate growing regions, especially on calcareous, P-fixing soils (Holloway et al., 2001). In such a region, young maize plants were found to accumulate greater quantities of P under SFZM (ridge tillage) than under uniform tillage (chisel plow), which was due to greater mycorrhizal activity in the ridge (McGonigle et al., 1990; McGonigle and Miller, 1993, 1996). Based on more recent studies of mycorrhizal P delivery to a variety of plant species, increased P delivery may result from increases in the abundance of Diversisporaceae (formerly Gigasporaceae). This family of AMF develops more extensive soil hyphae and is more effective at delivering P to host plants than other AMF families (Glomeraceae and Acaulosporaceae; Powell et al., 2009). Tillage strongly hinders Diversisporaceae activity (Verbruggen and Kiers, 2010), but the targeted disturbance of ridge top removal and later reformation (Figure 1) likely enables them to persist in ridge tillage systems (Ewing et al., unpublished).
In addition to fungi and bacteria, soil fauna may be better protected in SFZM systems. Soil fauna contribute to important agroecosystem services, including decomposition, nutrient cycling, bioturbation, and pest suppression (Coleman et al., 2004; Birkhofer et al., 2008; Suzuki et al., 2013). For example, soil macrofauna facilitate decomposition by fragmenting and redistributing plant residues in the soil profile (Brussaard et al., 2007; García-Palacios et al., 2013). It is well-established that tillage acts as a strong physical filter on soil faunal communities (Roger-Estrade et al., 2010). In a vegetable production system, the combination of reduced tillage (active turnover zone) and no-tillage (soil building zone) in SFZM strip tillage systems maintained higher earthworm and nematode populations compared to conventional, uniform tillage systems (Overstreet et al., 2010). Furthermore, when strip tillage was combined with strategic management of cover crop residues, predatory mite and collembolan (fungivore) densities and nematode community complexity increased compared to conventionally managed systems (Wang et al., 2011).
Nitrogen Cycling
Soil functional zone management systems may also enhance crop N nutrition by promoting greater synchrony between soil N availability and crop N requirements. Crop N demand varies over the growing season, and is greatest for row crops during vegetative growth (Olson and Kurtz, 1982; Robertson, 1997), which generally happens in mid- to late- summer. When fertilizer N is supplied at the time of planting, the resulting asynchrony with crop demand can encourage weed growth, lead to inefficient crop use of fertilizer, and drive N loss from soils via denitrification or leaching (Robertson, 1997; Crews and Peoples, 2005; Shanahan et al., 2008). These problems can be addressed by management that synchronizes N supply with peak crop N demand.
The key to N synchrony may be to manage N supply in both space and time (Shanahan et al., 2008). This is a central feature of SFZM, especially when redistribution of plant residues into the crop row is involved, such as under ridge tillage (John et al., 2004). Under a range of row crops and crop rotations, ridge tillage creates higher concentrations of soil organic C (SOC; Shi et al., 2012), potentially mineralizable N, microbial N (Müller et al., 2009b) and microbial biomass (Bezdicek et al., 2003; Grigera et al., 2007; Müller et al., 2009b) on the ridge-tops of crop rows compared with inter-rows. This spatial concentration of resources and microbial biomass leads to increased microbial activity in the crop row (Clay et al., 1995; Liebig et al., 1995; Müller et al., 2009a), and increases rates of N mineralization (Figure 2; Kane et al., 2015). Thus, ridge tillage appears to synchronize potentially mineralizable N supply with crop demand in both space and time, resulting in greater crop N uptake (Gordon et al., 1993; Kane et al., 2015). Similar increases in N mineralization have been observed in the inter-row spaces of strip tillage systems of both maize and orange trees (Citrus sinensis L.) Osbeck; Johnstone et al., 2009; Balota and Auler, 2011), but strip tillage was not found to improve N synchrony in a cabbage (Brassica oleracea L.) system (Haramoto and Brainard, 2012). This may indicate that the redistribution of plant and soil residues that occurs during ridge tillage is the key to unlocking the N synchrony potential of SFZM. Furthermore, to the extent that SFZM encourages nutrient recycling ecosystem services, then synchronized N can be supplied from internal sources (crop residue, cover crop, or weed residues), reducing the need for fertilizer inputs.
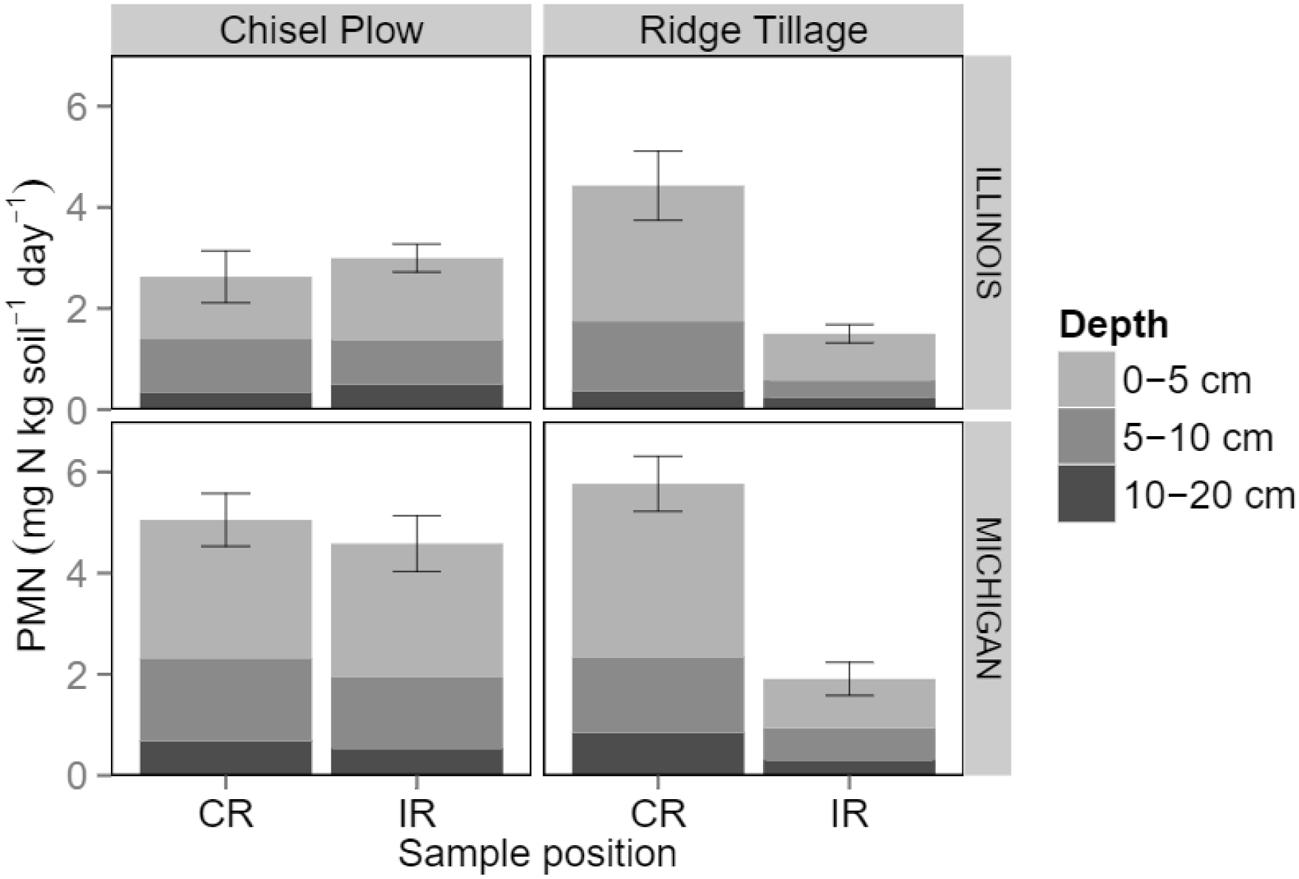
FIGURE 2. Potentially mineralizable N (PMN) at different depths and positions (CR: crop row; IR: inter-row) in two maize-soybean cropping systems during mid-summer. Error bars represent ± 1 SE. Adapted from Kane et al. (2015).
Potential Trade-Offs
Despite the wide range of benefits that may result from SFZM, undesirable effects may also arise, creating trade-offs associated with SFZM. Undesirable effects include the potential for increased populations of some pests due to less frequent and less intense tillage operations (Chaplin-Kramer et al., 2011). For example, incidence of Rhizoctonia root rot and parasitic nemadotes increased in no-tillage systems with residue retention compared with conventional tillage (Schroeder and Paulitz, 2006; Govaerts et al., 2007). However, when used in combination with other pest management practices, like diverse crop rotations, SFZM strategies that include an intra-seasonal tillage event, such as ridge tillage, can help disrupt pest populations while maintaining natural enemy populations (McKeown et al., 1998). Pruess et al. (1968) observed clustering of western corn rootworm (Diabrotica virgifera Le Conte) eggs in furrow positions and delayed larval development following an intra-seasonal ridging event. They suggested the ridging event relocated the previously uniformly dispersed eggs into the furrow while also burying the eggs under surface debris, lowering soil temperatures, and slowing larval development (Pruess et al., 1968). Additional research on the effects of timing of intra-seasonal tillage on pest and natural enemy populations will be necessary to further minimize pest management trade-offs associated with SFZM.
Regulating Soil Services
Soil Structure, Moisture, and Carbon Storage
The accumulation of SOM in agricultural systems has important implications for soil structure development (Bronick and Lal, 2005; Lal, 2009). SOM is a primary building block of aggregates – it serves to bind and stabilize soil micro-aggregates, which in turn coalesce to form macro-aggregates (Tisdall and Oades, 1982; Bronick and Lal, 2005; Lützow et al., 2006; Karami et al., 2012). Soil tillage and residue management affect aggregate development through their collective influence on SOM quality and accrual. Previous studies have found that SFZM systems increase organic matter (OM) in surface soil layers (0–15 cm) relative to conventional tillage (Angers et al., 1995; Unger, 1995). In turn, SFZM systems, much like no-tillage systems, have been found to increase aggregate stability and average size relative to conventional tillage (Kladivko et al., 1986; Mikha and Rice, 2004; Zibilske and Bradford, 2007). The relative improvements to soil structure in these studies were attributed to minimal tillage-induced disturbance to larger, more fragile aggregates.
The physical encapsulation of OM within soil aggregates plays an important role in the accumulation of soil C (Balesdent et al., 2000; Grandy and Robertson, 2007; Plaza et al., 2013). The OM contained within macro-aggregates is labile and particulate in nature, while micro-aggregate C is more stable, having undergone microbial processing (Elliot, 1986; Plaza et al., 2013; Zhang et al., 2013). Macro-aggregates are highly sensitive to management, with their stability depending largely on plant roots, fungal hyphae, tillage intensity, and microbial activity (Six et al., 2000; Rillig and Mummey, 2006; Zhang et al., 2013). In conventional systems, where macro-aggregate structures are regularly broken down, labile forms of C are released from physical protection resulting in rapid SOM depletion (Grandy and Robertson, 2006, 2007; Panettieri et al., 2015). The reduction in soil disturbance under SFZM increases soil aggregate formation, and the process of concentrating crop residues in inter-row positions has been found to increase concentrations of SOM (Unger, 1995).
The improvement of soil structure via enhanced aggregate formation under SFZM provides regulating services by facilitating rainfall infiltration and enhancing soil water holding capacity (Figure 3; Franzluebbers, 2002; Zibilske and Bradford, 2007). SFZM systems have been shown to conserve soil moisture more effectively than conventional tillage systems (Drury et al., 2006; Zibilske and Bradford, 2007; Williams et al., under review). This feature may be particularly important in terms of adapting agricultural systems to drought stress. Droughts are predicted to increase in frequency and severity with climate change (Gornall et al., 2010; Trenberth et al., 2014). No-tillage has been highlighted as a drought management option due to its ability to conserve soil moisture (Lal, 2004; Powlson et al., 2014). SFZM, because it features zones of no or reduced tillage, may therefore play a crucial role in buffering agricultural systems against drought, while minimizing trade-offs with provisioning services associated with no-tillage (Pittelkow et al., 2015). Put another way, SFZM may help build resilience to climate change while protecting long-term agricultural productivity.
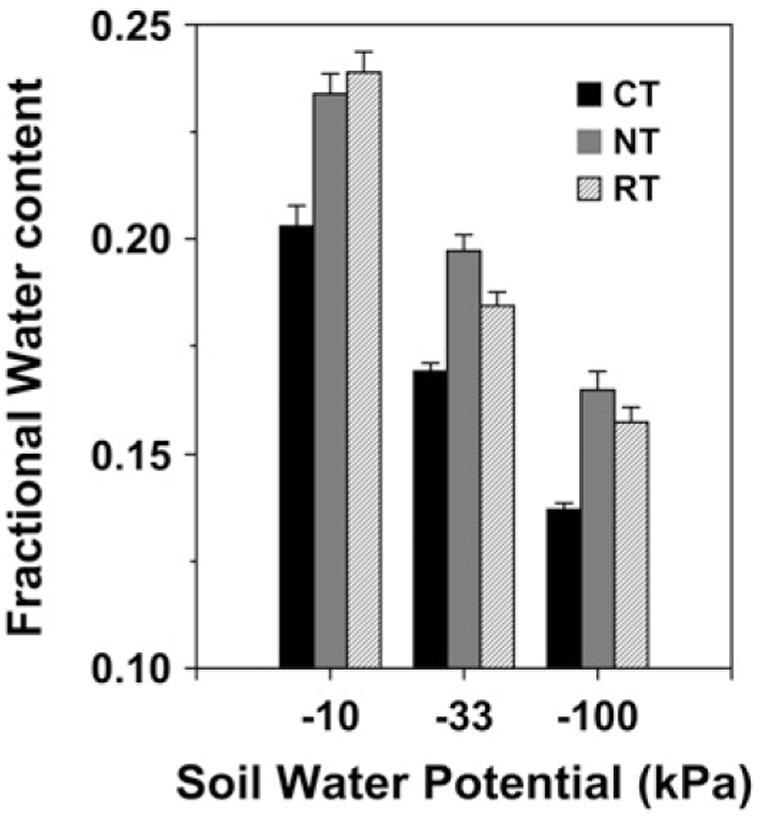
FIGURE 3. Water holding capacity at three water potentials in the top 2.5 cm of soil after 13 years of conventional tillage (CT), no-tillage (NT), and ridge tillage (RT). Error bars represent ± 1 SE. Reproduced from Zibilske and Bradford (2007).
In addition, we hypothesize that the heterogeneous soil environments created by SFZM allow development of greater fungal biomass by providing refugia from tillage disturbance (see Services Produced by Soil Biota above); fungal hyphae play an important role in the formation and stability of soil aggregates (Wilson et al., 2009; Peng et al., 2013; Lehmann and Rillig, 2015). Recent studies lend support to this hypothesis, as reduced tillage systems have been shown to promote greater fungal biomass and diversity relative to conventional tillage systems (van Groenigen et al., 2010; Säle et al., 2015). Furthermore, crops grown under ridge tillage have shown greater mycorrhizal colonization compared with crops grown under uniform tillage systems (McGonigle and Miller, 1993; McGonigle et al., 1999). Thus, by providing greater long-term protection of SOC by enhancing aggregate formation, SFZM could potentially reduce the release of CO2 and other greenhouse gasses back to the atmosphere, thereby helping to mitigate the contribution of agriculture to climate change.
Additional Regulating Services: The Case of Weed Control
Soil functional zone management may also provide regulating services that contribute to the suppression of weeds. Non-herbicidal weed suppression services will become increasingly valuable as populations of weeds that are resistant to glyphosate and other herbicides continue to become more abundant. The problem of herbicide resistant weeds is especially acute in conventional no-tillage systems, and particularly in those systems that rely on herbicide resistant crops, because of their exclusive reliance on herbicides for weed control (Mortensen et al., 2012). SFZM, through a variety of mechanisms, may reduce weed density and growth, shift the competitive balance from weeds to crops, and provide more opportunities for integrated weed management than conventional no-tillage or other uniformly managed systems.
One way that SFZM can contribute to the management of weeds is through promotion of AMF. AMF can suppress the development of both AMF host and non-host weed species (Jordan et al., 2000; Vatovec et al., 2005), thereby reducing crop yield losses to weeds (Rinaudo et al., 2010; Veiga et al., 2011). Several studies have found negative correlations between AM colonization and crop growth in no-tillage systems relative to conventional tillage, which has been attributed to cooler temperatures in no-tillage crop rows as a result of residue cover (McGonigle and Miller, 1996; McGonigle et al., 1999). SFZM may overcome such drawbacks by removing crop residues from crop rows and concentrating them in relatively undisturbed inter-rows (Figure 1). This uncoupling of soil temperatures and residues from areas of soil disturbance allows soil in row positions to warm more rapidly early in spring, while preserving an extensive AMF mycelial network for rapid root colonization in inter-rows (Johnson et al., 1997). Maize grown under ridge tillage has been shown to have increased mycorrhizal colonization and enhanced early season crop performance relative to no-tillage (Vivekanandan and Fixen, 1991; McGonigle and Miller, 1993). When AMF colonize multiple hosts they can increase nutrient transfer to the host that provides the most carbohydrates (Lekberg et al., 2010; Kiers et al., 2011). As such, by improving crop establishment and vigor relative to no-tillage, SFZM can alter interactions between crops and weeds via AMF, improving crop nutrition and performance, and inhibiting weed development. Such improvements have been demonstrated in a strip tillage system, where tomato (Solanum lycopersicum L.) performance was improved by AMF when in competition with bahiagrass (Paspalum notatum Flügge; Sylvia et al., 2001). However, further research is needed to quantify the contribution of AMF to weed suppression in addition to crop performance within SFZM systems.
Soil functional zone management may enhance weed suppression in other ways, particularly when integrated with cover crops. Cover crops present in inter-rows can suppress weeds through resource and light competition (Liebman and Dyck, 1993; Teasdale, 1996), disruption of weed life cycles (Moyer et al., 2000), physical suppression by cover crop residues (Moore et al., 1994), and release of phytotoxic chemicals (Kruidhof et al., 2009; Teasdale et al., 2012; Samedani et al., 2013). Release of phytotoxic chemicals from cover crop residues can also have negative effects on crop species (Kruidhof et al., 2011; Soltys et al., 2012), and this can be particularly true in uniform tillage systems. SFZM, particularly in ridge tillage systems, removes residues from the crop row and concentrates them in inter-row positions (Hatfield et al., 1998; Figure 1). Therefore, by actively managing the placement of phytotoxic cover crop residues, SFZM can minimize some of the potential trade-offs associated with the use of cover crops. The process of concentrating crop residues also promotes survival of soil pathogens in inter-row positions, by increasing inter-row soil moisture content (Cook and Haglund, 1991; Page et al., 2013; Manstretta and Rossi, 2015); weed seeds on or near the soil surface in inter-row positions are then subject to pathogen attack (Caesar, 2005), while crop seeds in the row avoid such attack.
The concentration of crop residues in inter-rows under SFZM may further control weeds by smothering and reducing light penetration to the soil, reducing weed emergence (Forcella and Lindstrom, 1988; Kruidhof et al., 2009). The re-ridging event in ridge tillage, where residues and soil are moved from the inter-row and concentrated on ridges (Figure 1), can also serve to smother weeds growing in the crop row (Buhler, 1992). The combination of concentrated crop residues and reduced thermal time accumulation in SFZM systems may provide an additional weed control mechanism.
SFZM and Provisioning Services
In our presentation of SFZM hitherto, we have sought to establish that improvements in soil regulating and supporting services can be achieved while maintaining existing levels of agricultural output. The successful integration of conventional, intensive agricultural management approaches with more environmentally sustainable practices such as no-tillage would represent a major advance in agronomy. However, given expected increases in global demand for food and other agricultural products by 2050 (Godfray et al., 2010; Tilman et al., 2011), and the need to limit conversion of additional lands to agriculture, it is not sufficient for the world’s existing crop production systems to maintain current levels of production; they must become more productive (Foley et al., 2011; Bommarco et al., 2013; Godfray and Garnett, 2014).
Temporal Intensification
One way of increasing the productivity of existing agricultural land is through temporal intensification, which aims to expand the annual time period in which harvestable crops are grown. Practices aimed at temporally intensifying agriculture are being increasingly implemented around the world (Ray and Foley, 2013). These include increasing crop harvest frequency per unit area and time by double or triple cropping (Heaton et al., 2013; Ray and Foley, 2013), and earlier planting of cultivars with longer maturation times (Sacks and Kucharik, 2011).
Temporal intensification may improve soil services by reducing or eliminating periods when soil is left bare or fallow. By replacing bare-soil fallows with live plant communities during some or all of the year, temporal intensification can provide a range of soil related regulating and supporting services, such as reduced rates of soil erosion and nutrient leaching (Dabney et al., 2001; Dean and Weil, 2009), increased microbial community size and activity (McDaniel et al., 2014; Tiemann et al., 2015), and weed suppression (Davis and Liebman, 2003; Carrera et al., 2004). In addition, temporal intensification provides opportunities to increase crop rotational diversity (Moore and Karlen, 2013). These factors enhance crop residue, root and exudate production, providing increased C resources for microbial processing (Kong et al., 2011; Tiemann et al., 2015), with subsequent soil quality benefits including long-term C storage and improved soil structure (Grandy and Robertson, 2007; Schmidt et al., 2011; Poeplau and Don, 2015; Tiemann et al., 2015).
Despite the potential benefits of temporal intensification, there are also large potential drawbacks, including reductions in the yields of each crop when multi-cropping is used for temporal intensification (Tonitto et al., 2006; Johnson et al., 2015). Such reductions may be severe if soil resources are exhausted or tied up by previous crops or their residues, or if harvest of one crop delays planting of the next crop. Such delays and the lack of operational flexibility they incur can severely limit production capacity. Other potential drawbacks include damage to soil structure from increases in soil cultivation intensity (Grandy and Robertson, 2006), and greater nutrient leaching and depletion of water resources due to increased fertilization and irrigation (Ju et al., 2009; Ray and Foley, 2013). Soil biodiversity may also be reduced by temporal intensification due to the deleterious effects of increased soil cultivation and elevated input of agrochemicals (Helgason et al., 1998; Mäder et al., 2002). Loss of soil biodiversity may curtail ecosystem functions that generate soil ecosystem services (Bardgett, 2005).
To mitigate these potential downsides while still realizing the inherent benefits of temporal intensification, novel management systems are needed. These systems must enable increases in the amount of product that can be extracted over a given time period while simultaneously protecting soil functional biodiversity and building soil quality. We contend that SFZM is a particularly promising strategy for achieving sustainable temporal intensification because it involves the creation of functionally distinct yet complementary soil zones. Through the integration of conventional, intensive management and reduced tillage practices, these zones are optimized for crop productivity (active turnover zone) and soil protection (soil building zone).
Dynamics of SFZM: Potential for a Virtuous Cycle Linking Yield and Soil Quality
We base our hypothesis of joint enhancement in provisioning and other ecosystem services via SFZM on a virtuous cycle model that links above-ground and below-ground processes (Figure 4). Specifically, we propose that SFZM engenders a self-reinforcing feedback process that couples improvements in soil regulating and supporting services (below-ground cycle) with improvements in provisioning services via increased field working days (above-ground cycle).
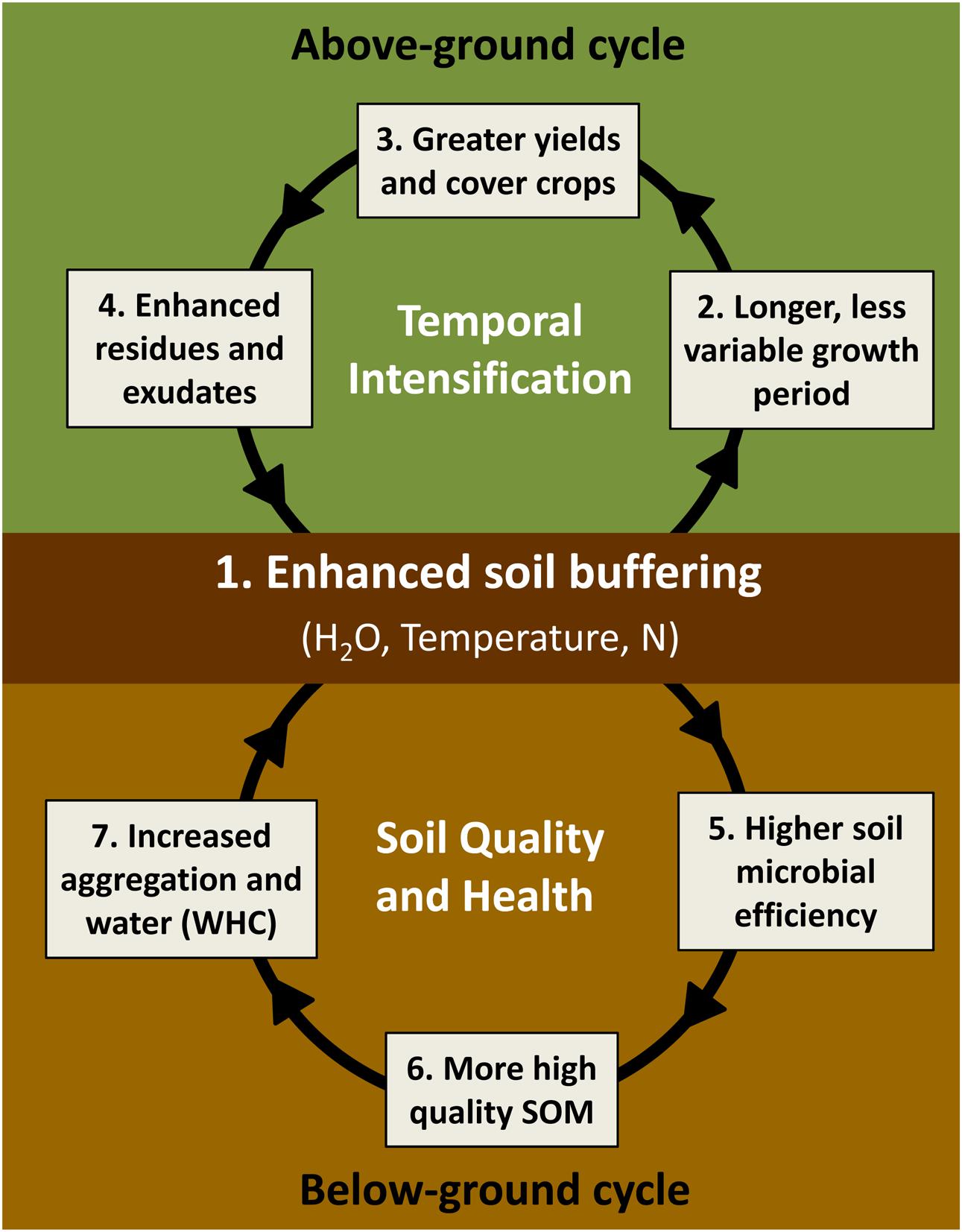
FIGURE 4. Proposed ‘virtuous cycles’ of SFZM. SFZM improves soil hydrothermal and fertility properties (buffering) (1), enabling earlier crop planting and a longer, more stable growth period, even in the face of variable weather patterns (2). This extended growing season supports greater yields from double cropping, crop residue harvest, and more effective cover crop production (3). An extended period of living plant cover enhances crop residue, root, and exudate production (4), resulting in higher soil microbial efficiencies (5) that drive the conversion of residues and microbial biomass into SOM (6). These biologically derived organic matter inputs improve soil quality and health by increasing aggregation, water holding capacity, and plant-available nutrients (7), which together confer and reinforce the soil’s capacity to buffer against variability in rainfall and temperature (1).
Above-Ground Processes in the Virtuous Cycle
A key component of sustainable temporal intensification is increasing the period of time during which crops can be grown and harvested on existing agricultural land. In real terms, this translates into a need for increased field working days, which can be achieved by enabling earlier soil cultivation and planting, by supporting crop growth later in the season, or by a combination of both.
Existing SFZM systems (e.g., ridge and strip tillage), which remove crop residues from crop row positions prior to planting, have been demonstrated to produce seedbed environments that warm and dry rapidly in early spring (Hatfield et al., 1998; Licht and Al-Kaisi, 2005). These seedbeds have similar hydrothermal properties to conventional tillage systems, which in turn have improved hydrothermal properties relative to no-tillage systems, i.e., are warmer and drier, resulting in improved seedling emergence relative to no-tillage (Cox et al., 1990; Kovar et al., 1992; Dwyer et al., 2000). Planting date has a large influence on crop productivity, and delays in planting due to climate fluctuations can severely reduce yields (Deryng et al., 2014). On poorly drained, finely textured soils, or during periods of excessive rainfall, ridge tillage can also improve seedbed hydrothermal conditions above that of conventional tillage, leading to earlier planting, greater accumulation of thermal time and improved yields (Cox et al., 1990; Eckert, 1990; Fausey, 1990). This provides the basis for an important premise of the virtuous cycle model (Figure 4): that SFZM increases field working days by allowing cultivation and planting to occur earlier in the season compared to when these operations could occur, for example, in an adjacent field managed with no-tillage approaches. SFZM would also likely outperform conventional tillage in terms of field working days in poorly drained soils or in years with wet springs (Figure 4, points 1 and 2).
Soil functional zone management can also extend the growing season by continuing to support crop growth later in the season. Existing SFZM systems concentrate soil moisture into crop inter-row positions (Müller et al., 2009b; Shi et al., 2012), substantially increasing soil moisture above that of conventional systems and maintaining it at levels similar or equivalent to no-tillage (Drury et al., 2003, 2006). These moisture-rich inter-rows may provide an important water resource during critical periods of crop development (Alvarez and Steinbach, 2009). Thus, by altering soil hydrothermal properties, SFZM can increase field working days at both ends of the growing season; allowing soil to be cultivated and/or planted earlier in the season, and maintaining soil moisture in inter-rows that can sustain crop growth later in the season or support planting of winter double crops. In other words, SFZM creates functionally distinct zones that together provide greater soil buffering to climate variability; SFZM buffers against extremes in soil temperature and moisture, and thereby provides a longer, less variable growth period (Figure 4, points 1 and 2).
The extension of the growing season afforded by SFZM enables greater utilization of solar radiation both at the beginning and end of the growing season, particularly in northern temperate regions. Longer seasons also allow greater capture of light energy and accumulation of hydrothermal time for both summer and winter crops in double cropping systems, increasing yield potential (Chen et al., 2011; Figure 4, point 3). The conservation of soil moisture through late summer in SFZM would also provide a water resource for the establishment of winter crops in double cropping systems, which are currently hampered by growing season duration. By extending the growing season, SFZM has the potential to reduce risks of seasonal crop yield reductions due to delayed harvest under temporal intensification. In addition, the ability of SFZM to enhance soil water conservation could potentially reduce requirements for additional irrigation, as required in some temporally intensified systems (Ray and Foley, 2013).
Temporal intensification may itself also help agriculture become more resilient to climate change. For example, double cropping, facilitated by SFZM, may shift phenologies of some crops, enabling them to avoid peak summer temperatures during critical development phases, when excessive heat can cause severe yield reductions (Seifert and Lobell, 2015). Moreover, SFZM may be particularly suited to support the production-enhancing aspect of temporal intensification because of new technologies for utilizing agricultural biomass from crop residues, and winter-annual cover crops. In the past, biomass crops and crop residues did not contribute to the food supply; however, a variety of new technologies now enable conversion of this biomass into a wide range of foodstuffs for direct and indirect human consumption, as well as biomass feedstocks for bioenergy and bioproducts (Chen and Zhang, 2015). In addition, by enhancing prospects for temporal intensification, SFZM may help reduce the conflict between food and biofuel production by enabling double cropping, potentially supplying both biofuels and food from the same field in the same season (Dale et al., 2010; Figure 4, point 3).
Below-Ground Processes in the Virtuous Cycle
By enabling an extension to the period of living plant cover, SFZM can also promote increases in the production of root exudates and crop residues (Figure 4, point 4). At the most basic level, the production of microbial biomass is governed largely by input quality and microbial physiological traits, such as microbial C-use efficiency (Sinsabaugh et al., 2013; Wieder et al., 2014, 2015). Root exudates and plant residues are primary sources of these C inputs, and drive microbial activity, biomass and community composition (Rasse et al., 2005; Hartmann et al., 2009; Rousk and Frey, 2015). Root exudates, in particular, are highly labile, and contain more reduced C compounds and lower C:N ratios, encouraging higher microbial C-use efficiency (Manzoni et al., 2012). Microbial activity is reduced by periods of sustained soil moisture deficiency (Borken and Matzner, 2009), causing reductions in soil nutrient availability (Emmett et al., 2004; Larsen et al., 2011). In addition, repeated wet-dry cycling leads to pulses of soil C and N mineralization, potentially accelerating SOM mineralization over time (Borken and Matzner, 2009). This diminishes soil water holding capacity and increases susceptibility to future soil moisture deficits. Thus, management that produces improved conditions for microbial growth (e.g., adequate water and temperature, plus greater quantities of root exudates), as can be achieved by SFZM, may sustain greater microbial activity and efficiency, thereby enhancing nutrient turnover processes (Figure 4, point 5).
Traditional soil models suggest that it is not possible to maintain soil quality under conditions of intensifying production and greater extraction of soil resources, because removal of crop residues and intensification of tillage and fertilization will deplete SOM (Janzen, 2006; Grandy and Robertson, 2007). This may not be the case in agroecosystems managed to create distinct soil functional zones. Existing SFZM systems, such as ridge tillage, have been found to be similar to no-tillage systems in that they support greater microbial biomass than conventionally tilled systems (Angers et al., 1992; Müller et al., 2009b; Zhang et al., 2013). Emerging experimental and theoretical evidence shows that dead microbial biomass (i.e., necromass) is a significant fraction of SOM (Grandy and Neff, 2008; Schmidt et al., 2011; Cotrufo et al., 2013; Frey et al., 2013; Wieder et al., 2014). The continuous and rapid turnover of living microbial biomass can produce, over time, a considerable amount of necromass (Liang and Balser, 2011), which stabilizes SOM (Simpson et al., 2007; Schmidt et al., 2011; Miltner et al., 2012; Cotrufo et al., 2013; Gleixner, 2013; Figure 4, point 6).
Although microbial biomass can be rapidly mineralized by soil organisms due to its favorable energy yield and low C:N ratio (Blagodatskaya et al., 2014), microbial necromass and other microbial by-products can also be selectively preserved via interactions with soil minerals and incorporation into soil aggregates (Lützow et al., 2006; Throckmorton et al., 2015; Figure 4, point 7). In fact, microbial necromass, metabolites, and decomposition products account for the majority of stabilized SOM (Simpson et al., 2007; Grandy and Neff, 2008; Kleber and Johnson, 2010; Schmidt et al., 2011). The accumulation of stabilized SOM within soil aggregates in turn improves infiltration of precipitation and increases soil water holding capacity (Franzluebbers, 2002; Zibilske and Bradford, 2007; Figure 4, point 7). By encouraging the development of greater microbial biomass, SFZM may halt declines of SOM observed under conventional tillage, and instead contribute positively to SOM accumulation and soil structure development while simultaneously supporting greater yield extraction through temporal intensification.
Concluding Remarks
Development and implementation of novel agroecological management systems that allow increases in provisioning services (yield) while simultaneously enhancing regulating and supporting ecosystem services are urgently needed. As our review shows, SFZM offers a strategy for integrating the production benefits associated with intensively tilled field crop production systems with the soil ecosystem service benefits associated with no-tillage. In short, SFZM offers the potential to achieve the best of both approaches. The soil heterogeneity produced by SFZM enhances soil functional biodiversity, and allows farmers to harness this biodiversity to elicit desirable ecosystem functions at appropriate times and places. This can lead to greater resource-use efficiency and closer synchrony between soil processes and crop physiological demands. Moreover, the ability of SFZM to favorably alter soil hydrothermal properties allows extension of the growing season, both at the beginning and end. This opens opportunities for increasing agricultural production via temporal intensification. Coupled with improvements to soil regulating and supporting services, SFZM therefore offers a vehicle for optimizing multiple ecosystem goods and services in agricultural systems.
Widespread adoption and refinement of SFZM depends on progress on several fronts. Further research on all aspects of SFZM systems will be required to ensure that service delivery can be optimized to meet specific needs of farmers and society in particular cropping systems and geographies. As well, progress on adoption and refinement of SFZM systems is likely to be strongly affected by societal demand for the full range of regulating and supporting ecosystem services that such systems may be able to provide (Mitchell et al., 2016). The case of ridge tillage in maize-soybean production in central North America is instructive: despite its economic viability (Archer et al., 2002), this form of SFZM is not widely used in the US. In this region, it appears that the perceived value of ecosystem services resulting from ridge tillage do not provide a sufficient incentive for its widespread adoption. However, new incentives are appearing, such as the rapidly growing interest in management systems that promote “soil health” (Lehman et al., 2015), increasing innovation in incentives for agricultural soil C storage (Funk et al., 2015), and more stringent demands for nutrient-use efficiency and other ecosystem services from sustainability-oriented supply chains (Davidson et al., 2014). If there is significant societal demand for the full range of ecosystem services from SFZM, the collective ingenuity of farmers and agricultural engineers can be expected to drive rapid development and implementation of SFZM. This is evidenced by the widespread adoption of zonal tillage techniques in the Central Valley region of California (USA) in response to imperatives to improve resource-use efficiency and environmental performance of production systems in this region (Mitchell et al., 2016).
Author Contributions
All authors contributed to the manuscript by reviewing literature, discussing and developing ideas, writing text sections and revising drafts of the manuscript. AW, DK, AD, AG, SH, MH, RK, DM, RS, SS, KS, AY, and NJ developed the initial idea for the manuscript and all contributed to framing and general writing (Abstract, Introduction, SFZM, SFZM and ecosystem services, SFZM and provisioning services, Concluding remarks). PE, LA, and AY contributed to SFZM and Regulating soil services. AJ, ML, and YL contributed to Supporting soil services. AW, AD, AG, RS, and NJ contributed to Virtuous cycles. AW and PE developed Figure 1; AG and RS developed Figure 4.
Conflict of Interest Statement
The authors declare that the research was conducted in the absence of any commercial or financial relationships that could be construed as a potential conflict of interest.
Acknowledgments
This project was supported by an Agriculture and Food Research Initiative (AFRI) Climate Change Mitigation and Adaptation in Agriculture grant (2011-67003-30343), from the United States Department of Agriculture (USDA) National Institute of Food and Agriculture (NIFA). The use of trade, firm, or corporation names in this paper is for the information and convenience of the reader. Such use does not constitute an official endorsement or approval by the USDA or the Agricultural Research Service of any product or service to the exclusion of others that may be suitable. USDA is an equal opportunity provider and employer.
References
Alvarez, R., and Steinbach, H. S. (2009). A review of the effects of tillage systems on some soil physical properties, water content, nitrate availability and crops yield in the Argentine Pampas. Soil Tillage Res. 104, 1–15. doi: 10.1016/j.still.2009.02.005
Angers, D. A., N’dayegamiye, A., and Côté, D. (1992). Tillage-induced differences in organic matter of particle-size fractions and microbial biomass. Soil Sci. Soc. Am. J. 57, 512–516. doi: 10.2136/sssaj1993.03615995005700020035x
Angers, D. A., Voroney, R. P., and Côté, D. (1995). Dynamics of soil organic matter and corn residues affected by tillage practices. Soil Sci. Soc. Am. J. 59, 1311–1315. doi: 10.2136/sssaj1995.03615995005900050016x
Archer, D. W., Pikul, J. L. Jr., and Riedell, W. E. (2002). Economic risk, returns and input use under ridge and conventional tillage in the northern Corn Belt, USA. Soil Tillage Res. 67, 1–8. doi: 10.1016/S0167-1987(02)00016-8
Balesdent, J., Chenu, C., and Balabane, M. (2000). Relationship of soil organic matter dynamics to physical protection and tillage. Soil Biol. Biochem. 53, 215–230. doi: 10.1016/s0167-1987(99)00107-5
Balota, E. L., and Auler, P. A. M. (2011). Soil carbon and nitrogen mineralization under different tillage systems and permanent groundcover cultivation between orange trees. Revista Brasil. Fruticult. 33, 637–648. doi: 10.1590/S0100-29452011005000071
Balota, E. L., Calegari, A., Nakatani, A. S., and Coyne, M. S. (2014). Benefits of winter cover crops and no-tillage for microbial parameters in a Brazilian Oxisol: a long-term study. Agric. Ecosyst. Environ. 197, 31–40. doi: 10.1016/j.agee.2014.07.010
Bardgett, R. D. (2005). The Biology of Soil: A Community and Ecosystem Approach. Oxford: Oxford University Press. doi: 10.1093/acprof:oso/9780198525035.001.0001
Baveye, P. C., Rangel, D., Jacobson, A. R., Laba, M., Darnault, C., Otten, W., et al. (2011). From Dust Bowl to Dust Bowl: soils are still very much a frontier of science. Soil Sci. Soc. Am. J. 75, 2037–2048. doi: 10.2136/sssaj2011.0145
Bezdicek, D. F., Beaver, T., and Granatstein, D. (2003). Subsoil ridge tillage and lime effects on soil microbial activity, soil pH, erosion, and wheat and pea yield in the Pacific Northwest, USA. Soil Tillage Res. 74, 55–63. doi: 10.1016/S0167-1987(03)00091-6
Birkhofer, K., Bezemer, T. M., Bloem, J., Bonkowski, M., Christensen, S., Dubois, D., et al. (2008). Long-term organic farming fosters below and aboveground biota: implications for soil quality, biological control and productivity. Soil Biol. Biochem. 40, 2297–2308. doi: 10.1016/j.soilbio.2008.05.007
Blagodatskaya, E., Blagodatsky, S., Anderson, T.-H., and Kuzyakov, Y. (2014). Microbial growth and carbon use efficiency in the rhizosphere and root-free soil. PLoS ONE 9:e93282. doi: 10.1371/journal.pone.0093282
Bommarco, R., Kleijn, D., and Potts, S. G. (2013). Ecological intensification: harnessing ecosystem services for food security. Trends Ecol. Evol. 28, 230–238. doi: 10.1016/j.tree.2012.10.012
Borken, W., and Matzner, E. (2009). Reappraisal of drying and wetting effects on C and N mineralization and fluxes in soils. Glob. Change Biol. 15, 808–824. doi: 10.1111/j.1365-2486.2008.01681.x
Bronick, C. J., and Lal, R. (2005). Soil structure and management: a review. Geoderma 124, 3–22. doi: 10.1016/j.geoderma.2004.03.005
Brussaard, L., De Ruiter, P. C., and Brown, G. G. (2007). Soil biodiversity for agricultural sustainability. Agric. Ecosyst. Environ. 121, 233–244. doi: 10.1016/j.agee.2006.12.013
Buhler, D. D. (1992). Population dynamics and control of annual weeds in corn (Zea mays) as influenced by tillage systems. Weed Sci. 40, 241–248.
Caesar, A. (2005). Melding ecology, classical weed biocontrol, and plant microbial ecology can inform improved practices in controlling invasive plant species. Biol. Control 35, 240–246. doi: 10.1016/j.biocontrol.2005.06.001
Carrera, L. M., Abdul-Baki, A. A., and Teasdale, J. R. (2004). Cover crop management and weed suppression in no-tillage sweet corn production. HortScience 39, 1262–1266.
Chaplin-Kramer, R., O’rourke, E., Blitzer, E. J., and Kremen, C. (2011). A meta-analysis of crop pest and natrual enemy response to landscape complexity. Ecol. Lett. 14, 922–932. doi: 10.1111/j.1461-0248.2011.01642.x
Chen, H.-G., and Zhang, Y.-H. P. (2015). New biorefineries and sustainable agriculture: increased food, biofuels, and ecosystem security. Renew. Sustain. Energy Rev. 47, 117–132. doi: 10.1016/j.rser.2015.02.048
Chen, X.-P., Cui, Z.-L., Vitousek, P. M., Cassman, K. G., Matson, P. A., Bai, J.-S., et al. (2011). Integrated soil-crop system management for food security. Proc. Natl. Acad. Sci. U.S.A. 108, 6399–6404. doi: 10.1073/pnas.1101419108
Clay, D. E., Schumacher, T. E., and Brix-Davis, K. A. (1995). Carbon and nitrogen mineralization in row and interrow areas of chisel and ridge tillage systems. Soil Tillage Res. 35, 167–174. doi: 10.1016/0167-1987(95)00484-X
Coleman, D. C., Crossley, D. A. Jr., and Hendrix, P. F. (2004). Fundamentals of Soil Ecology. Burlington, MA: Elsevier Academic Press.
Cook, R. J., and Haglund, W. A. (1991). Wheat yield depression associated with conservation tillage caused by root pathogens in the soil not phytotoxins from the straw. Soil Biol. Biochem. 23, 1125–1132. doi: 10.1016/0038-0717(91)90024-E
Cotrufo, M. F., Wallenstein, M. D., Boot, C. M., Denef, K., and Paul, E. (2013). The Microbial Efficiency-Matrix Stabilization (MEMS) framework integrates plant litter decomposition with soil organic matter stabilization: do labile plant inputs form stable soil organic matter? Glob. Change Biol. 19, 988–995. doi: 10.1111/gcb.12113
Cox, W. J., Zobel, R. W., Van Es, H. M., and Otis, D. J. (1990). Growth development and yield of maize under three tillage systems in the northeastern USA. Soil Tillage Res. 18, 295–310. doi: 10.1016/0167-1987(90)90067-N
Crews, T. E., and Peoples, M. B. (2005). Can the synchrony of nitrogen supply and crop demand be improved in legume and fertilizer-based agroecosystems? A review. Nutr. Cycling Agroecosyst. 72, 101–120. doi: 10.1007/s10705-004-6480-1
Crowther, T. W., Sokol, N. W., Oldfield, E. E., Maynard, D. S., Thomas, S. M., and Bradford, M. A. (2015). Environmental stress response limits microbial necromass contributions to soil organic carbon. Soil Biol. Biochem. 85, 153–161. doi: 10.1016/j.soilbio.2015.03.002
Culman, S. W., Young-Mathews, A., Hollander, A. D., Ferris, H., Sánchez-Moreno, S., O’green, A. T., et al. (2010). Biodiversity is associated with indicators of soil ecosystem functions over a landscape gradient of agricultural intensification. Landsc. Ecol. 25, 1333–1348. doi: 10.1007/s10980-010-9511-0
Dabney, S. M., Delgado, J. A., and Reeves, D. W. (2001). Using winter cover crops to improve soil and water quality. Commun. Soil Sci. Plant Anal. 32, 1221–1250. doi: 10.1081/CSS-100104110
Dale, B. E., Bals, B. D., Kim, S., and Eranki, P. (2010). Biofuels done right: land efficient animal feeds enable large environmental and energy benefits. Environ. Sci. Technol. 44, 8385–8389. doi: 10.1021/es101864b
Davidson, E. A., Galloway, J. N., Millar, N., and Leach, A. M. (2014). N-related greenhouse gases in North America: innovations for a sustainable future. Curr. Opin. Environ. Sustain. 9, 1–8. doi: 10.1016/j.cosust.2014.07.003
Davis, A. S., and Liebman, M. (2003). Cropping system effects on giant foxtail (Setaria faberi) demography: I. Green manure and tillage timing. Weed Sci. 51, 919–929. doi: 10.1614/P2002-133A
de Vries, F. T., Hoffland, E., Van Eekeren, N., Brussaard, L., and Bloem, J. (2006). Fungal/bacterial ratios in grasslands with contrasting nitrogen management. Soil Biol. Biochem. 38, 2092–2103. doi: 10.1016/j.soilbio.2006.01.008
Dean, J. E., and Weil, R. R. (2009). Brassica cover crops for nitrogen retention in the mid-Atlantic Coastal Plain. J. Environ. Qual. 38, 520–528. doi: 10.2134/jeq2008.0066
Deryng, D., Conway, D., Ramankutty, N., Price, J., and Warren, R. (2014). Global crop yield response to extreme heat stress under multiple climate change futures. Environ. Res. Lett. 9:034011. doi: 10.1088/1748-9326/9/3/034011
Drury, C. F., Reynolds, W. D., Tan, C. S., Welacky, T. W., Calder, W., and Mclaughlin, N. B. (2006). Emissions of nitrous oxide and carbon dioxide: influence of tillage type and nitrogen placement depth. Soil Sci. Soc. Am. J. 70, 570–581. doi: 10.2136/sssaj2005.0042
Drury, C. F., Tan, C. S., Reynolds, W. D., Welacky, T. W., Weaver, S. E., Hamill, A. S., et al. (2003). Impacts of zone tillage and red clover on corn performance and soil physical quality. Soil Sci. Soc. Am. J. 67, 867–877. doi: 10.2136/sssaj2003.0867
Dwyer, L. M., Ma, B. L., De Jong, R., and Tollenaar, M. (2000). Assessing corn seedbed conditions for emergence. Can. J. Soil Sci. 80, 53–61. doi: 10.4141/S99-003
Eckert, D. J. (1990). Ridge planting for row crops on a poorly drained soil. 1. Rotation and drainage effects. Soil Tillage Res. 18, 181–188. doi: 10.1016/0167-1987(90)90058-L
Elliot, E. T. (1986). Aggregate structure and carbon, nitrogen, and phosphorus in native and cultivated soils. Soil Sci. Soc. Am. J. 50, 627–633. doi: 10.2136/sssaj1986.03615995005000030017x
Emmett, B. A., Beier, C., Estiarte, M., Tietema, A., Kristensen, H. L., Williams, D., et al. (2004). The response of soil processes to climate change: results from manipulation studies of shrublands across an environmental gradient. Ecosystems 7, 625–637. doi: 10.1007/s10021-004-0220-x
Ettema, C. H., and Wardle, D. A. (2002). Spatial soil ecology. Trends Ecol. Evol. 17, 177–183. doi: 10.1016/S0169-5347(02)02496-5
Fausey, N. R. (1990). Experience with ridge-till on slowly permeable soils in Ohio. Soil Tillage Res. 18, 195–205. doi: 10.1016/0167-1987(90)90060-Q
Foley, J. A., Ramankutty, N., Brauman, K. A., Cassidy, E. S., Gerber, J. S., Johnston, M., et al. (2011). Solutions for a cultivated planet. Nature 478, 337–342. doi: 10.1038/nature10452
Forcella, F., and Lindstrom, M. J. (1988). Weed seed populations in ridge and conventional tillage. Weed Sci. 36, 500–503.
Franzluebbers, A. J. (2002). Water infiltration and soil structure related to organic matter and its stratification with depth. Soil Tillage Res. 66, 197–205. doi: 10.1016/S0167-1987(02)00027-2
Frey, S. D., Elliott, E. T., and Paustian, K. (1999). Bacterial and fungal abundance and biomass in conventional and no-tillage agroecosystems along two climatic gradients. Soil Biol. Biochem. 31, 573–585. doi: 10.1016/S0038-0717(98)00161-8
Frey, S. D., Elliott, E. T., Paustian, K., and Peterson, G. A. (2000). Fungal translocation as a mechanism for soil nitrogen inputs to surface residue decomposition in a no-tillage agroecosystem. Soil Biol. Biochem. 32, 689–698. doi: 10.1016/S0038-0717(99)00205-9
Frey, S. D., Lee, J., Melillo, J. M., and Six, J. (2013). The temperature response of soil microbial efficiency and its feedback to climate. Nat. Clim. Change 3, 395–398. doi: 10.1038/nclimate1796
Frey, S. D., Six, J., and Elliott, E. T. (2003). Reciprocal transfer of carbon and nitrogen by decomposer fungi at the soil–litter interface. Soil Biol. Biochem. 35, 1001–1004. doi: 10.1016/S0038-0717(03)00155-X
Funk, R., Pascual, U., Joosten, H., Duffy, C., Pan, G., La Scala, N., et al. (2015). “From potential to implementation: an innovation framework to realize the benefits of soil carbon,” in Soil Carbon: Science, Management and Policy for Multiple Benefits, eds S. A. Banwart, E. Noellemeyer and E. Milne (Wallingford: CAB International).
García-Palacios, P., Maestre, F. T., Kattge, J., and Wall, D. H. (2013). Climate and litter quality differently modulate the effects of soil fauna on litter decomposition across biomes. Ecol. Lett. 16, 1045–1053. doi: 10.1111/ele.12137
Giller, K. E., Witter, E., Corbeels, M., and Tittonell, P. (2009). Conservation agriculture and smallholder farming in Africa: the heretics’ view. Field Crops Res. 14, 23–34. doi: 10.1016/j.fcr.2009.06.017
Gleixner, G. (2013). Soil organic matter dynamics: a biological perspective derived from the use of compound-specific isotopes studies. Ecol. Res. 28, 683–695. doi: 10.1007/s11284-012-1022-9
Godfray, H. C. J., Beddington, J. R., Crute, I. R., Haddad, L., Lawrence, D., Muir, J. F., et al. (2010). Food security: the challenge of feeding 9 billion people. Science 327, 812–818. doi: 10.1126/science.1185383
Godfray, H. C. J., and Garnett, T. (2014). Food security and sustainable intensification. Philos. Trans. R. Soc. B 369, 20120273. doi: 10.1098/rstb.2012.0273
Gordon, W. B., Rickerl, D. H., Sorensen, D. R., and Wieland, P. K. (1993). Tillage and nitrogen effects on growth, nitrogen content, and yield of corn. Commun. Soil Sci. Plant Anal. 24, 421–441. doi: 10.1080/00103629309368812
Gornall, J., Betts, R., Burke, E., Clark, R., Camp, J., Willett, K., et al. (2010). Implications of climate change for agricultural productivity in the early twenty-first century. Philos. Trans. R. Soc. B 365, 2973–2989. doi: 10.1098/rstb.2010.0158
Govaerts, B., Fuentes, M., Mezzalama, M., Nicol, J. M., Deckers, J., Etchevers, J. D., et al. (2007). Infiltration, soil moisture, root rot and nematode populations after 12 years of different tillage, residue and crop rotation managements. Soil Tillage Res. 94, 209–219. doi: 10.1016/j.still.2006.07.013
Grandy, A. S., and Neff, J. C. (2008). Molecular C dynamics downstream: the biochemical decomposition sequence and its impact on soil organic matter structure and function. Sci. Total Environ. 404, 297–307. doi: 10.1016/j.scitotenv.2007.11.013
Grandy, A. S., and Robertson, G. P. (2006). Aggregation and organic matter protection following tillage of a previously uncultivated soil. Soil Sci. Soc. Am. J. 70, 1398–1406. doi: 10.2136/sssaj2005.0313
Grandy, A. S., and Robertson, G. P. (2007). Land-use intensity effects on soil organic carbon accumulation rates and mechanisms. Ecosystems 10, 58–73. doi: 10.1007/s10021-006-9010-y
Grigera, M. S., Drijber, R. A., and Wienhold, B. J. (2007). Redistribution of crop residues during row cultivation creates a biologically enhanced environment for soil microorganisms. Soil Tillage Res. 94, 550–554. doi: 10.1016/j.still.2006.08.016
Haramoto, E. R., and Brainard, D. C. (2012). Strip tillage and oat cover crops increase soil moisture and influence N mineralization patterns in cabbage. HortScience 47, 1596–1602.
Hartmann, A., Schmid, M., Van Tuinen, D., and Berg, G. (2009). Plant-driven selection of microbes. Plant Soil 321, 235–257. doi: 10.1007/s11104-008-9814-y
Hatfield, J. L., Allmaras, R. R., Rehm, G. W., and Lowery, B. (1998). Ridge tillage for corn and soybean production: environmental quality impacts. Soil Tillage Res. 48, 145–154. doi: 10.1016/S0167-1987(98)00141-X
Heaton, E. A., Schulte, L. A., Berti, M., Langeveld, H., Zegada-Lizarazu, W., Parrish, D., et al. (2013). Managing a second-generation crop portfolio through sustainable intensification: examples from the USA and the EU. Biofuels Bioprod. Bioref. 7, 702–714. doi: 10.1002/bbb.1429
Heenan, D. P., Chan, K. Y., and Knight, P. G. (2004). Long-term impact of rotation, tillage and stubble management on the loss of soil organic carbon and nitrogen from a Chromic Luvisol. Soil Tillage Res. 76, 59–68. doi: 10.1016/j.still.2003.08.005
Helgason, T., Daniell, T. J., Husband, R., Fitter, A. H., and Young, J. P. W. (1998). Ploughing up the wood-wide web? Nature 394:431. doi: 10.1038/28764
Hendrix, P. F., Parmelee, R. W., Crossley, D. A. Jr., Coleman, D. C., Odum, E. P., and Groffman, P. M. (1986). Detritus food webs in conventional and no-tillage agroecosystems. BioScience 36, 374–380. doi: 10.2307/1310259
Hobbs, P. R., Sayre, K., and Gupta, R. (2008). The role of conservation agriculture in sustainable agriculture. Philos. Trans. R. Soc. B 363, 543–555. doi: 10.1098/rstb.2007.2169
Hodge, A., and Storer, K. (2015). Arbuscular mycorrhiza and nitrogen: implications for individual plants through to ecosystems. Plant Soil 386, 1–19. doi: 10.1007/s11104-014-2162-1
Holloway, R. E., Bertrand, I., Frischke, A. J., Brace, D. M., Mclaughlin, M. J., and Shepperd, W. (2001). Improving fertiliser efficiency on calcareous and alkaline soils with fluid sources of P, N and Zn. Plant Soil 236, 209–219. doi: 10.1023/A:1012720909293
Janzen, H. H. (2006). The soil carbon dilemma: shall we hoard it or use it? Soil Biol. Biochem. 38, 419–424. doi: 10.1016/j.soilbio.2005.10.008
John, B., Ludwig, B., Potthoff, M., and Flessa, H. (2004). Carbon and nitrogen mineralization after maize harvest between and within maize rows: a microcosm study using 13C natural abundance. J. Plant Nutr. Soil Sci. 167, 270–276. doi: 10.1002/jpln.200321255
Johnson, G. A., Kantar, M. B., Betts, K. J., and Wyse, D. L. (2015). Field pennycress production and weed control in a double crop system with soybean in Minnesota. Agron. J. 107, 532–540. doi: 10.2134/agronj14.0292
Johnson, N. C., Graham, J. H., and Smith, F. A. (1997). Functioning of mycorrhizal associations along the mutualism-parasitism continuum. New Phytol. 135, 575–585. doi: 10.1046/j.1469-8137.1997.00729.x
Johnstone, P. R., Arnold, N., Pearson, A., and Parker, M. (2009). Alternative tillage practice for establishing maize silage and reducing soil nitrogen mineralisation. Agron. New Zealand 39, 23–32.
Jordan, N., Boody, G., Broussard, W., Glover, J. D., Keeney, D., Mccown, B. H., et al. (2007). Sustainable development of the agricultural bio-economy. Science 316, 1570–1571. doi: 10.1126/science.1141700
Jordan, N. R., Zhang, J., and Huerd, S. (2000). Arbuscular-mycorrhizal fungi: potential roles in weed management. Weed Res. 40, 397–410. doi: 10.1046/j.1365-3180.2000.00207.x
Ju, X.-T., Xing, G.-X., Chen, X.-P., Zhang, S.-L., Zhang, L.-J., Liu, X.-J., et al. (2009). Reducing environmental risk by improving N management in intensive Chinese agricultural systems. Proc. Natl. Acad. Sci. U.S.A. 106, 3041–3046. doi: 10.1073/pnas.0813417106
Kabir, Z. (2005). Tillage or no-tillage: impacts on mycorrhizae. Can. J. Plant Sci. 85, 23–29. doi: 10.4141/P03-160
Kane, D. A., Snapp, S. S., and Davis, A. S. (2015). Ridge tillage concentrates potentially mineralizable soil nitrogen, facilitating maize nitrogen uptake. Soil Sci. Soc. Am. J. 79, 81–88. doi: 10.2136/sssaj2014.07.0273
Karami, A., Homaee, M., Afzalinia, S., Ruhipour, H., and Basirat, S. (2012). Organic resource management: impacts on soil aggregate stability and other soil physico-chemical properties. Agric. Ecosyst. Environ. 148, 22–28. doi: 10.1016/j.agee.2011.10.021
Kiers, E. T., Duhamel, M., Beesetty, Y., Mensah, J. A., Franken, O., Verbruggen, E., et al. (2011). Reciprocal rewards stabilize cooperation in the mycorrhizal symbiosis. Science 333, 880–882. doi: 10.1126/science.1208473
Kladivko, E. J. (2001). Tillage systems and soil ecology. Soil Tillage Res. 61, 61–76. doi: 10.1016/S0167-1987(01)00179-9
Kladivko, E. J., Griffith, D. R., and Mannering, J. V. (1986). Conservation tillage effects on soil properties and yield of corn and soya beans in Indiana. Soil Tillage Res. 8, 277–287. doi: 10.1016/0167-1987(86)90340-5
Kleber, M., and Johnson, M. G. (2010). “Advances in understanding the molecular structure of soil organic matter: implications for interactions in the environment,” in Advances in Agronomy, ed. D. L. Sparks (Cambridge, MA: Academic Press), 77–142. doi: 10.1016/s0065-2113(10)06003-7
Kong, A. Y. Y., Scow, K. M., Córdova-Kreylos, A. L., Holmes, W. E., and Six, J. (2011). Microbial community composition and carbon cycling within soil microenvironments of conventional, low-input, and organic cropping systems. Soil Biol. Biochem. 43, 20–30. doi: 10.1016/j.soilbio.2010.09.005
Kovar, J. L., Barber, S. A., Kladivko, E. J., and Griffith, D. R. (1992). Characterization of soil temperature, water content, and maize root distribution in two tillage systems. Soil Tillage Res. 24, 11–27. doi: 10.1016/0167-1987(92)90069-N
Kremen, C., and Miles, A. (2012). Ecosystem services in biologically diversified versus conventional farming systems: benefits, externalities, and trade-offs. Ecol. Soc. 17, 40. doi: 10.5751/es-05035-170440
Kruidhof, H. M., Bastiaans, L., and Kropff, M. J. (2009). Cover crop residue management for optimizing weed control. Plant Soil 318, 169–184. doi: 10.1007/s11104-008-9827-6
Kruidhof, H. M., Gallandt, E. R., Haramoto, E. R., and Bastiaans, L. (2011). Selective weed suppression by cover crop residues: effects of seed mass and timing of species’ sensitivity. Weed Res. 51, 177–186. doi: 10.1111/j.1365-3180.2010.00825.x
Lal, R. (2004). Soil carbon sequestration impacts on global climate change and food security. Science 304, 1623–1627. doi: 10.1126/science.1097396
Lal, R. (2009). Challenges and opportunities in soil organic matter research. Eur. J. Soil Sci. 60, 158–169. doi: 10.1111/j.1365-2389.2008.01114.x
Lal, R. (2015). “Challenges and opportunities in precision agriculture,” in Soil-Specific Farming: Precision Agriculture, eds R. Lal and B. A. Stewart (Boca Raton, FL: CRC Press), 391–400. doi: 10.1201/b18759-17
Larsen, K. S., Andresen, L. C., Beier, C., Jonasson, S., Albert, K. R., Ambus, P. E. R., et al. (2011). Reduced N cycling in response to elevated CO2, warming, and drought in a Danish heathland: synthesizing results of the CLIMAITE project after two years of treatments. Glob. Change Biol. 17, 1884–1899. doi: 10.1111/j.1365-2486.2010.02351.x
Lehman, R. M., Acosta-Martinez, V., Buyer, J. S., Cambardella, C. A., Collins, H. P., Ducey, T. F., et al. (2015). Soil biology for resilient, healthy soil. J. Soil Water Conserv. 70, 12A–18A. doi: 10.2489/jswc.70.1.12a
Lehmann, A., and Rillig, M. C. (2015). Understanding mechanisms of soil biota involvement in soil aggregation: a way forward with saprobic fungi? Soil Biol. Biochem. 88, 298–302. doi: 10.1016/j.soilbio.2015.06.006
Lekberg, Y., Hammer, E. C., and Olsson, P. A. (2010). Plants as resource islands and storage units - adopting the mycocentric view of arbuscular mycorrhizal networks. FEMS Microbiol. Ecol. 74, 336–345. doi: 10.1111/j.1574-6941.2010.00956.x
Liang, C., and Balser, T. C. (2011). Microbial production of recalcitrant organic matter in global soils: implications for productivity and climate policy. Nat. Rev. Microbiol. 9, 75. doi: 10.1038/nrmicro2386-c1
Licht, M. A., and Al-Kaisi, M. (2005). Strip-tillage effect on seedbed soil temperature and other soil physical properties. Soil Tillage Res. 80, 233–249. doi: 10.1016/j.still.2004.03.017
Liebig, M. A., Jones, A. J., Doran, J. W., and Mielke, L. N. (1995). Potential soil respiration and relationship to soil properties in ridge tillage. Soil Sci. Soc. Am. J. 59, 1430–1435. doi: 10.2136/sssaj1995.03615995005900050032x
Liebman, M., and Dyck, E. (1993). Crop rotation and intercropping strategies for weed management. Ecol. Appl. 3, 92–122. doi: 10.2307/1941795
Ludwig, M., Achtenhagen, J., Miltner, A., Eckhardt, K.-U., Leinweber, P., Emmerling, C., et al. (2015). Microbial contribution to SOM quantity and quality in density fractions of temperate arable soils. Soil Biol. Biochem. 81, 311–322. doi: 10.1016/j.soilbio.2014.12.002
Lützow, M. V., Kögel-Knabner, I., Ekschmitt, K., Matzner, E., Guggenberger, G., Marschner, B., et al. (2006). Stabilization of organic matter in temperate soils: mechanisms and their relevance under different soil conditions – a review. Eur. J. Soil Sci. 57, 426–445. doi: 10.1111/j.1365-2389.2006.00809.x
Mäder, P., Fließbach, A., Dubois, D., Gunst, L., Fried, P., and Niggli, U. (2002). Soil fertility and biodiversity in organic farming. Science 296, 1694–1697. doi: 10.1126/science.1071148
Mannering, J. V., and Fenster, C. R. (1983). What is conservation tillage? J. Soil Water Conserv. 38, 140–143.
Manstretta, V., and Rossi, V. (2015). Modelling the effect of weather on moisture fluctuations in maize stalk residues, an important inoculum source for plant diseases. Agric. For. Meteorol. 207, 83–93. doi: 10.1016/j.agrformet.2015.04.001
Manzoni, S., Taylor, P., Richter, A., Porporato, A., and Ågren, G. I. (2012). Environmental and stoichiometric controls on microbial carbon-use efficiency in soils. New Phytol. 196, 79–91. doi: 10.1111/j.1469-8137.2012.04225.x
Martens, D. A. (2001). “Nitrogen cycling under different soil management systems,” in Advances in Agronomy, ed. D. L. Sparks (Cambridge, MA: Academic Press), 143–192.
McCormick, K., and Kautto, N. (2013). The bioeconomy in Europe: an overview. Sustainability 5, 2589–2608. doi: 10.3390/su5062589
McDaniel, M. D., Tiemann, L. K., and Grandy, A. S. (2014). Does agricultural crop diversity enhance soil microbial biomass and organic matter dynamics? A meta-analysis. Ecol. Appl. 24, 560–570. doi: 10.1890/13-0616.1
McGonigle, T. P., Evans, D. G., and Miller, M. H. (1990). Effect of degree of soil disturbance on mycorrhizal colonization and phosphorous absorption by maize in growth chamber and field experiments. New Phytol. 116, 629–636. doi: 10.1111/j.1469-8137.1990.tb00548.x
McGonigle, T. P., and Miller, M. H. (1993). Mycorrhizal development and phosphorus absorption in maize under conventional and reduced tillage. Soil Sci. Soc. Am. J. 57, 1002–1006. doi: 10.2136/sssaj1993.03615995005700040020x
McGonigle, T. P., and Miller, M. H. (1996). Mycorrhizae, phosphorus absorption, and yield of maize in response to tillage. Soil Sci. Soc. Am. J. 60, 1856–1861. doi: 10.2136/sssaj1996.03615995006000060034x
McGonigle, T. P., Miller, M. H., and Young, D. (1999). Mycorrhizae, crop growth, and crop phosphorus nutrition in maize-soybean rotations given various tillage treatments. Plant Soil 210, 33–42. doi: 10.1023/A:1004633512450
McKeown, A. W., Cerkauskas, R. F., Potter, J. W., and Driel, L. V. (1998). Long-term evaluation of cover crop and strip-tillage on tomato yield, foliar diseases and nematode populations. Can. J. Plant Sci. 78, 341–348. doi: 10.4141/P97-090
Mikha, M. M., and Rice, C. W. (2004). Tillage and manure effects on soil and aggregate-associated carbon and nitrogen. Soil Sci. Soc. Am. J. 68, 809–816. doi: 10.2136/sssaj2004.8090
Miltner, A., Bombach, P., Schmidt-Brücken, B., and Kästner, M. (2012). SOM genesis: microbial biomass as a significant source. Biogeochemistry 111, 41–55. doi: 10.1007/s10533-011-9658-z
Mitchell, J. P., Carter, L. M., Reicosky, D. C., Shrestha, A., Pettygrove, G. S., Klonsky, K. M., et al. (2016). A history of tillage in California’s Central Valley. Soil Tillage Res. 157, 52–64. doi: 10.1016/j.still.2015.10.015
Moore, K. J., and Karlen, D. L. (2013). Double cropping opportunities for biomass crops in the north central USA. Biofuels 4, 605–615. doi: 10.4155/bfs.13.50
Moore, M. J., Gillespie, T. J., and Swanton, C. J. (1994). Effect of cover crop mulches on weed emergence, weed biomass, and soybean (Glycine max) development. Weed Technol. 8, 512–518.
Mortensen, D. A., Egan, J. F., Maxwell, B. D., Ryan, M. R., and Smith, R. G. (2012). Navigating a critical juncture for sustainable weed management. BioScience 62, 75–84. doi: 10.1525/bio.2012.62.1.12
Moyer, J. R., Blackshaw, R. E., Smith, E. G., and Mcginn, S. M. (2000). Cereal cover crops for weed suppression in a summer fallow-wheat cropping sequence. Can. J. Plant Sci. 80, 441–449. doi: 10.4141/P99-099
Müller, E., Wildhagen, H., Quintern, M., Heß, J., Wichern, F., and Joergensen, R. G. (2009a). CO2 evolution from a ridge tilled and a mouldboard ploughed Luvisol in the field. Appl. Soil Ecol. 43, 89–94. doi: 10.1016/j.apsoil.2009.06.005
Müller, E., Wildhagen, H., Quintern, M., Heß, J., Wichern, F., and Joergensen, R. G. (2009b). Spatial patterns of soil biological and physical properties in a ridge tilled and a ploughed Luvisol. Soil Tillage Res. 105, 88–95. doi: 10.1016/j.still.2009.05.011
Olson, R. A., and Kurtz, L. T. (1982). “Crop nitrogen requirements, utilization and fertilization,” in Nitrogen in Agricultural Soils, ed. F. J. Stephenson (Madison, WI: American Society of Agronomy), 567–604.
Overstreet, L. F., Hoyt, G. D., and Imbriani, J. (2010). Comparing nematode and earthworm communities under combinations of conventional and conservation vegetable production practices. Soil Tillage Res. 110, 42–50. doi: 10.1016/j.still.2010.06.009
Page, K., Dang, Y., and Dalal, R. (2013). Impacts of conservation tillage on soil quality, including soil-borne crop diseases, with a focus on semi-arid grain cropping systems. Austral. Plant Pathol. 42, 363–377. doi: 10.1007/s13313-013-0198-y
Palm, C., Blanco-Canqui, H., Declerck, F., Gatere, L., and Grace, P. (2014). Conservation agriculture and ecosystem services: an overview. Agric. Ecosyst. Environ. 187, 87–105. doi: 10.1016/j.agee.2013.10.010
Panettieri, M., Berns, A. E., Knicker, H., Murillo, J. M., and Madejón, E. (2015). Evaluation of seasonal variability of soil biogeochemical properties in aggregate-size fractioned soil under different tillages. Soil Tillage Res. 151, 39–49. doi: 10.1016/j.still.2015.02.008
Peng, S., Guo, T., and Liu, G. (2013). The effects of arbuscular mycorrhizal hyphal networks on soil aggregations of purple soil in southwest China. Soil Biol. Biochem. 57, 411–417. doi: 10.1016/j.soilbio.2012.10.026
Pittelkow, C. M., Liang, X., Linquist, B. A., Van Groenigen, K. J., Lee, J., Lundy, M. E., et al. (2015). Productivity limits and potentials of the principles of conservation agriculture. Nature 517, 365–368. doi: 10.1038/nature13809
Plaza, C., Courtier-Murias, D., Fernández, J. M., Polo, A., and Simpson, A. J. (2013). Physical, chemical, and biochemical mechanisms of soil organic matter stabilization under conservation tillage systems: a central role for microbes and microbial by-products in C sequestration. Soil Biol. Biochem. 57, 124–134. doi: 10.1016/j.soilbio.2012.07.026
Poeplau, C., and Don, A. (2015). Carbon sequestration in agricultural soils via cultivation of cover crops - a meta-analysis. Agric. Ecosyst. Environ. 200, 33–41. doi: 10.1016/j.agee.2014.10.024
Postma-Blaauw, M. B., De Goede, R. G. M., Bloem, J., Faber, J. H., and Brussaard, L. (2010). Soil biota community structure and abundance under agricultural intensification and extensification. Ecology 91, 460–473. doi: 10.1890/09-0666.1
Postma-Blaauw, M. B., De Goede, R. G. M., Bloem, J., Faber, J. H., and Brussaard, L. (2012). Agricultural intensification and de-intensification differentially affect taxonomic diversity of predatory mites, earthworms, enchytraeids, nematodes and bacteria. Appl. Soil Ecol. 57, 39–49. doi: 10.1016/j.apsoil.2012.02.011
Powell, J. R., Parrent, J. L., Hart, M. M., Klironomos, J. N., Rillig, M. C., and Maherali, H. (2009). Phylogenetic trait conservatism and the evolution of functional trade-offs in arbuscular mycorrhizal fungi. Proc. R. Soc. Lond. B 276, 4237–4245. doi: 10.1098/rspb.2009.1015
Powlson, D. S., Stirling, C. M., Jat, M. L., Gerard, B. G., Palm, C. A., Sanchez, P. A., et al. (2014). Limited potential of no-till agriculture for climate change mitigation. Nat. Clim. Change 4, 678–683. doi: 10.1038/nclimate2292
Pruess, K. P., Weekman, G. T., and Somerhalder, B. R. (1968). Western corn rootworm egg distribution and adult emergence under two corn tillage systems. J. Econ. Entomol. 61, 1424–1427. doi: 10.1093/jee/61.5.1424
Rasse, D. P., Rumpel, C., and Dignac, M.-F. (2005). Is soil carbon mostly root carbon? Mechanisms for a specific stabilisation. Plant Soil 269, 341–356. doi: 10.1007/s11104-004-0907-y
Ray, D. K., and Foley, J. A. (2013). Increasing global crop harvest frequency: recent trends and future directions. Environ. Res. Lett. 8:044041. doi: 10.1088/1748-9326/8/4/044041
Rillig, M. C., and Mummey, D. L. (2006). Mycorrhizas and soil structure. New Phytol. 171, 41–53. doi: 10.1111/j.1469-8137.2006.01750.x
Rinaudo, V., Bàrberi, P., Giovannetti, M., and Van Der Heijden, M. G. A. (2010). Mycorrhizal fungi suppress aggressive agricultural weeds. Plant Soil 333, 7–20. doi: 10.1007/s11104-009-0202-z
Robertson, G. P. (1997). “Nitrogen use efficiency in row-crop agriculture: crop nitrogen use and soil nitrogen loss,” in Ecology in Agriculture, ed. L. E. Jackson (San Diego, CA: Academic Press), 347–365.
Roger-Estrade, J., Anger, C., Bertrand, M., and Richard, G. (2010). Tillage and soil ecology: partners for sustainable agriculture. Soil Tillage Res. 111, 33–40. doi: 10.1016/j.still.2010.08.010
Rousk, J., and Frey, S. D. (2015). Revisiting the hypothesis that fungal-to-bacterial dominance characterizes turnover of soil organic matter and nutrients. Ecol. Monogr. 85, 457–472. doi: 10.1890/14-1796.1
Sacks, W. J., and Kucharik, C. J. (2011). Crop management and phenology trends in the U.S. Corn Belt: impacts on yields, evapotranspiration and energy balance. Agric. For. Meteorol. 151, 882–894. doi: 10.1016/j.agrformet.2011.02.010
Säle, V., Aguilera, P., Laczko, E., Mäder, P., Berner, A., Zihlmann, U., et al. (2015). Impact of conservation tillage and organic farming on the diversity of arbuscular mycorrhizal fungi. Soil Biol. Biochem. 84, 38–52. doi: 10.1016/j.soilbio.2015.02.005
Samedani, B., Juraimi, A. S., Anwar, M. P., Rafii, M. Y., Awadz, S. A. S., and Anuar, A. R. (2013). Phytotoxic effects of Pueraria javanica litter on growth of weeds Asystasia gangetica and Pennisetum polystachion. Allelopathy J. 32, 191–202.
Schimel, J. P., and Bennett, J. (2004). Nitrogen mineralization: challenges of a changing paradigm. Ecology 85, 591–602. doi: 10.1890/03-8002
Schmidt, M. W. I., Torn, M. S., Abiven, S., Dittmar, T., Guggenberger, G., Janssens, I. A., et al. (2011). Persistence of soil organic matter as an ecosystem property. Nature 478, 49–56. doi: 10.1038/nature10386
Schroeder, K. L., and Paulitz, T. C. (2006). Root diseases of wheat and barley during the transition from conventional tillage to direct seeding. Plant Dis. 90, 1247–1253. doi: 10.1094/PD-90-1247
Seifert, C. A., and Lobell, D. B. (2015). Response of double cropping suitability to climate change in the United States. Environ. Res. Lett. 10:024002. doi: 10.1088/1748-9326/10/2/024002
Shanahan, J. F., Kitchen, N. R., Raun, W. R., and Schepers, J. S. (2008). Responsive in-season nitrogen management for cereals. Comput. Electron. Agric. 61, 51–62. doi: 10.1016/j.compag.2007.06.006
Shi, X. H., Yang, X. M., Drury, C. F., Reynolds, W. D., Mclaughlin, N. B., and Zhang, X. P. (2012). Impact of ridge tillage on soil organic carbon and selected physical properties of a clay loam in southwestern Ontario. Soil Tillage Res. 120, 1–7. doi: 10.1016/j.still.2012.01.003
Simpson, A. J., Simpson, M. J., Smith, E., and Kelleher, B. P. (2007). Microbially derived inputs to soil organic matter: are current estimates too low? Environ. Sci. Technol. 41, 8070–8076. doi: 10.1021/es071217x
Sinsabaugh, R. L., Manzoni, S., Moorhead, D. L., and Richter, A. (2013). Carbon use efficiency of microbial communities: stoichiometry, methodology and modelling. Ecol. Lett. 16, 930–939. doi: 10.1111/ele.12113
Six, J., Elliot, E. T., and Paustian, K. (2000). Soil macroaggregate turnover and microaggregate formation: a mechanism for C sequestration under no-tillage agriculture. Soil Biol. Biochem. 32, 2099–2103. doi: 10.1016/S0038-0717(00)00179-6
Six, J., Frey, S. D., Thiet, R. K., and Batten, K. M. (2006). Bacterial and fungal contributions to carbon sequestration in agroecosystems. Soil Sci. Soc. Am. J. 70, 555–569. doi: 10.2136/sssaj2004.0347
Soltys, D., Bogatek, R., and Gniazdowska, A. (2012). Phytotoxic effects of cyanamide on seed germination and seedling growth of weed and crop species. Acta Biol. Cracov. Ser. Bot. 54, 87–92. doi: 10.2478/v10182-012-0025-8
Strickland, M. S., and Rousk, J. (2010). Considering fungal:bacterial dominance in soils – methods, controls, and ecosystem implications. Soil Biol. Biochem. 42, 1385–1395. doi: 10.1016/j.soilbio.2010.05.007
Suzuki, Y., Grayston, S. J., and Prescott, C. E. (2013). Effects of leaf litter consumption by millipedes (Harpaphe haydeniana) on subsequent decomposition depends on litter type. Soil Biol. Biochem. 57, 116–123. doi: 10.1016/j.soilbio.2012.07.020
Sylvia, D. M., Alagely, A. K., Chellemi, D. O., and Demchenko, L. W. (2001). Arbuscular mycorrhizal fungi influence tomato competition with bahiagrass. Biol. Fertil. Soils 34, 448–452. doi: 10.1007/s00374-001-0429-1
Teasdale, J. R. (1996). Contribution of cover crops to weed management in sustainable agricultural systems. J. Prod. Agric. 9, 475–479. doi: 10.2134/jpa1996.0475
Teasdale, J. R., Rice, C. P., Cai, G., and Mangum, R. W. (2012). Expression of allelopathy in the soil environment: soil concentration and activity of benzoxazinoid compounds released by rye cover crop residue. Plant Ecol. 213, 1893–1905. doi: 10.1007/s11258-012-0057-x
Throckmorton, H., Bird, J., Monte, N., Doane, T., Firestone, M., and Horwath, W. (2015). The soil matrix increases microbial C stabilization in temperate and tropical forest soils. Biogeochemistry 122, 35–45. doi: 10.1007/s10533-014-0027-6
Tiemann, L. K., Grandy, A. S., Atkinson, E. E., Marin-Spiotta, E., and Mcdaniel, M. D. (2015). Crop rotational diversity enhances belowground communities and functions in an agroecosystem. Ecol. Lett. 18, 761–771. doi: 10.1111/ele.12453
Tilman, D., Balzer, C., Hill, J., and Befort, B. L. (2011). Global food demand and the sustainable intensification of agriculture. Proc. Natl. Acad. Sci. U.S.A. 108, 20260–20264. doi: 10.1073/pnas.1116437108
Tilman, D., Cassman, K. G., Matson, P. A., Naylor, R., and Polasky, S. (2002). Agricultural sustainability and intenstive production practices. Nature 418, 671–677. doi: 10.1038/nature01014
Tisdall, J. M., and Oades, J. M. (1982). Organic matter and water-stable aggregates in soils. J. Soil Sci. 33, 141–163. doi: 10.1111/j.1365-2389.1982.tb01755.x
Tonitto, C., David, M. B., and Drinkwater, L. E. (2006). Replacing bare fallows with cover crops in fertilizer-intensive cropping systems: a meta-analysis of crop yield and N dynamics. Agric. Ecosyst. Environ. 112, 58–72. doi: 10.1016/j.agee.2005.07.003
Trenberth, K. E., Dai, A., Van Der Schrier, G., Jones, P. D., Barichivich, J., Briffa, K. R., et al. (2014). Global warming and changes in drought. Nat. Clim. Change 4, 17–22. doi: 10.1038/nclimate2067
Treseder, K. K., and Lennon, J. T. (2015). Fungal traits that drive ecosystem dynamics on land. Microbiol. Mol. Biol. Rev. 79, 243–262. doi: 10.1128/MMBR.00001-15
Unger, P. W. (1995). Organic matter and water-stable aggregate distribution in ridge-tilled surface soil. Soil Sci. Soc. Am. J. 59:1141. doi: 10.2136/sssaj1995.03615995005900040028x
van der Heijden, M. G. A., Bardgett, R. D., and Van Straalen, N. M. (2008). The unseen majority: soil microbes as drivers of plant diversity and productivity in terrestrial ecosystems. Ecol. Lett. 11, 296–310. doi: 10.1111/j.1461-0248.2007.01139.x
van Groenigen, K.-J., Bloem, J., Bååth, E., Boeckx, P., Rousk, J., Bodé, S., et al. (2010). Abundance, production and stabilization of microbial biomass under conventional and reduced tillage. Soil Biol. Biochem. 42, 48–55. doi: 10.1016/j.soilbio.2009.09.023
Vatovec, C., Jordan, N., and Huerd, S. (2005). Responsiveness of certain agronomic weed species to arbuscular mycorrhizal fungi. Renew. Agric. Food Syst. 20, 181–189. doi: 10.1079/RAF2005115
Veiga, R. S. L., Jansa, J., Frossard, E., and Van Der Heijden, M. G. A. (2011). Can arbuscular mycorrhizal fungi reduce the growth of agricultural weeds? PLoS ONE 6:e27825. doi: 10.1371/journal.pone.0027825
Verbruggen, E., and Kiers, E. T. (2010). Evolutionary ecology of mycorrhizal functional diversity in agricultural systems. Evol. Appl. 3, 547–560. doi: 10.1111/j.1752-4571.2010.00145.x
Verbruggen, E., Van Der Heijden, M. G. A., Weedon, J. T., Kowalchuk, G. A., and Röling, W. F. M. (2012). Community assembly, species richness and nestedness of arbuscular mycorrhizal fungi in agricultural soils. Mol. Ecol. 21, 2341–2353. doi: 10.1111/j.1365-294X.2012.05534.x
Vivekanandan, M., and Fixen, P. E. (1991). Cropping systems effects on mycorrhizal colonization, early growth, and phosphorus uptake of corn. Soil Sci. Soc. Am. J. 55, 136–140. doi: 10.2136/sssaj1991.03615995005500010024x
Wang, K. H., Hooks, C. R. R., and Marahatta, S. P. (2011). Can using a strip-tilled cover cropping system followed by surface mulch practice enhance organisms higher up in the soil food web hierarchy? Appl. Soil Ecol. 49, 107–117. doi: 10.1016/j.apsoil.2011.06.008
Wardle, D. A. (1995). Impacts of disturbance on detritus food webs in agro-ecosystems of contrasting tillage and weed management practices. Adv. Ecol. Res. 26, 105–185. doi: 10.1016/S0065-2504(08)60065-3
Wieder, W. R., Grandy, A. S., Kallenbach, C. M., and Bonan, G. B. (2014). Integrating microbial physiology and physio-chemical principles in soils with the MIcrobial-MIneral Carbon Stabilization (MIMICS) model. Biogeosciences 11, 3899–3917. doi: 10.5194/bg-11-3899-2014
Wieder, W. R., Grandy, A. S., Kallenbach, C. M., Taylor, P. G., and Bonan, G. B. (2015). Representing life in the Earth system with soil microbial functional traits in the MIMICS model. Geosci. Model Dev. Discuss. 8, 2011–2052. doi: 10.5194/gmdd-8-2011-2015
Wilson, G. W. T., Rice, C. W., Rillig, M. C., Springer, A., and Hartnett, D. C. (2009). Soil aggregation and carbon sequestration are tightly correlated with the abundance of arbuscular mycorrhizal fungi: results from long-term field experiments. Ecol. Lett. 12, 452–461. doi: 10.1111/j.1461-0248.2009.01303.x
Zhang, S., Li, Q., Lü, Y., Zhang, X., and Liang, W. (2013). Contributions of soil biota to C sequestration varied with aggregate fractions under different tillage systems. Soil Biol. Biochem. 62, 147–156. doi: 10.1016/j.soilbio.2013.03.023
Keywords: crop yield, ecosystem services, precision tillage, soil biodiversity, soil management, temporal intensification, trade-offs, zonal tillage
Citation: Williams A, Kane DA, Ewing PM, Atwood LW, Jilling A, Li M, Lou Y, Davis AS, Grandy AS, Huerd SC, Hunter MC, Koide RT, Mortensen DA, Smith RG, Snapp SS, Spokas KA, Yannarell AC and Jordan NR (2016) Soil Functional Zone Management: A Vehicle for Enhancing Production and Soil Ecosystem Services in Row-Crop Agroecosystems. Front. Plant Sci. 7:65. doi: 10.3389/fpls.2016.00065
Received: 30 August 2015; Accepted: 14 January 2016;
Published: 05 February 2016.
Edited by:
Karl Ritz, University of Nottingham, UKReviewed by:
Tapan Kumar Adhya, KIIT University, IndiaBlanca B. Landa, Spanish National Research Council – Institute for Sustainable Agriculture, Spain
Copyright © 2016 Williams, Kane, Ewing, Atwood, Jilling, Li, Lou, Davis, Grandy, Huerd, Hunter, Koide, Mortensen, Smith, Snapp, Spokas, Yannarell and Jordan. This is an open-access article distributed under the terms of the Creative Commons Attribution License (CC BY). The use, distribution or reproduction in other forums is permitted, provided the original author(s) or licensor are credited and that the original publication in this journal is cited, in accordance with accepted academic practice. No use, distribution or reproduction is permitted which does not comply with these terms.
*Correspondence: Alwyn Williams, YWx3eW4ud2lsbGlhbXNAb3V0bG9vay5jb20=