- 1Sugarcane Research Institute, Guangxi Academy of Agricultural Sciences/Key Laboratory of Sugarcane Biotechnology and Genetic Improvement (Guangxi), Ministry of Agriculture and Rural Affairs/Guangxi Key Laboratory of Sugarcane Genetic Improvement, Nanning, Guangxi, China
- 2Department of Botany, Mohanlal Sukhadia University, Udaipur, Rajasthan, India
- 3Nuclear Agriculture and Biotechnology Division, Bhabha Atomic Research Centre, Mumbai, MH, India
- 4Homi Bhabha National Institute, Mumbai, MH, India
- 5College of Agriculture, Jawaharlal Nehru Krishi Vishwa Vidyalaya, Ganj Basoda, Vidisha, Madhya Pradesh, India
- 6Dr. Rajendra Prasad Central Agricultural University, Pusa, Samastipur, Bihar, India
- 7Department of Life Sciences and Biological Sciences, IES University, Bhopal, Madhya Pradesh, India
- 8Plant Cytogenetics and Molecular Biology Group, Faculty of Natural Sciences, Institute of Biology, Biotechnology and Environmental Protection, University of Silesia in Katowice, Katowice, Poland
- 9Department of Botany, University of Delhi, Delhi, India
Sustainable food security and safety are major concerns on a global scale, especially in developed nations. Adverse agroclimatic conditions affect the largest agricultural-producing areas, which reduces the production of crops. Achieving sustainable food safety is challenging because of several factors, such as soil flooding/waterlogging, ultraviolet (UV) rays, acidic/sodic soil, hazardous ions, low and high temperatures, and nutritional imbalances. Plant growth-promoting rhizobacteria (PGPR) are widely employed in in-vitro conditions because they are widely recognized as a more environmentally and sustainably friendly approach to increasing crop yield in contaminated and fertile soil. Conversely, the use of nanoparticles (NPs) as an amendment in the soil has recently been proposed as an economical way to enhance the texture of the soil and improving agricultural yields. Nowadays, various research experiments have combined or individually applied with the PGPR and NPs for balancing soil elements and crop yield in response to control and adverse situations, with the expectation that both additives might perform well together. According to several research findings, interactive applications significantly increase sustainable crop yields more than PGPR or NPs alone. The present review summarized the functional and mechanistic basis of the interactive role of PGPR and NPs. However, this article focused on the potential of the research direction to realize the possible interaction of PGPR and NPs at a large scale in the upcoming years.
Introduction
Recently, soil amendments have been implemented in agroecosystems to promote plant growth and development, especially by adding organic and inorganic nutrients. Adding specific substances to the soil enhances its capacity to sustain plant life (Urra et al., 2019; Rubin et al., 2023; Kumari et al., 2023b). Plant growth-promoting rhizobacteria (PGPR) and nanomaterials (NMs) or nanoparticles (NPs) are under consideration as a novel approach to boost crop productivity and soil fertility (El-Ramady et al., 2022; Upadhayay et al., 2023; Rajput et al., 2023a; Kumari et al., 2024). Several research studies have demonstrated numerous benefits that soil organic amendments may provide, including better soil texture, higher soil fertility, restored soil health, and, ultimately, higher crop yield (Urra et al., 2019; Rani et al., 2023).
Because of the serious risk factors to human health caused by antibiotic-resistant bacteria, antibiotic residues, and antibiotic-resistant genes that exist in agricultural organic amendments, such contaminants in emerging pollutants are still a major problem (Upadhayay et al., 2023). Soil supplements should have attributes including ecological safeguards and negatively affect the fertility of the soil, soil composition, or the enviro-ecosystem (Garbowski et al., 2022; Rajput et al., 2023a). Owing to their unique properties, PGPRs, and NPs have attracted more interest in recent years as potential soil amendments that can mitigate the risk associated with other soil additions under normal and adverse conditions (Chen et al., 2021; Tolisano and Del Buono, 2023; Kumari et al., 2024).
Most PGPRs have been reported and confirmed to improve plant productivity by reducing environmental challenges (Singh et al., 2021; Kumari et al., 2023a). Through both direct and indirect strategies, it may enhance the overall quality of soil and plant yield (Figure 1) (Sharma et al., 2023; Rajput et al., 2023a). The functions of PGPR occur through direct mechanisms such as nitrogen fixation, phosphate, and potassium solubilization, and the production of growth-promoting phytohormones like indole acetic acid (IAA) and siderophores. However, indirect mechanisms are associated with the production of lytic enzymes and antibiotics, dropping the soil pH and producing exopolysaccharides. Several studies have assessed the efficacy of PGPR for maintaining a sustainable agroecosystem in normal and stressful conditions (Oleńska et al., 2020; Magnabosco et al., 2023; Timofeeva et al., 2023). Numerous articles and meta-analyses have observed the beneficial impacts of NPs on soil health and agronomic productivity as well as the variables that facilitate the ameliorative role of NPs (Urra et al., 2019; Sharma et al., 2020; Verma et al., 2022a, b). The NPs have also alleviated various environmental stresses during plant development (Dilnawaz et al., 2023; Pramanik et al., 2023; Verma et al., 2023a).
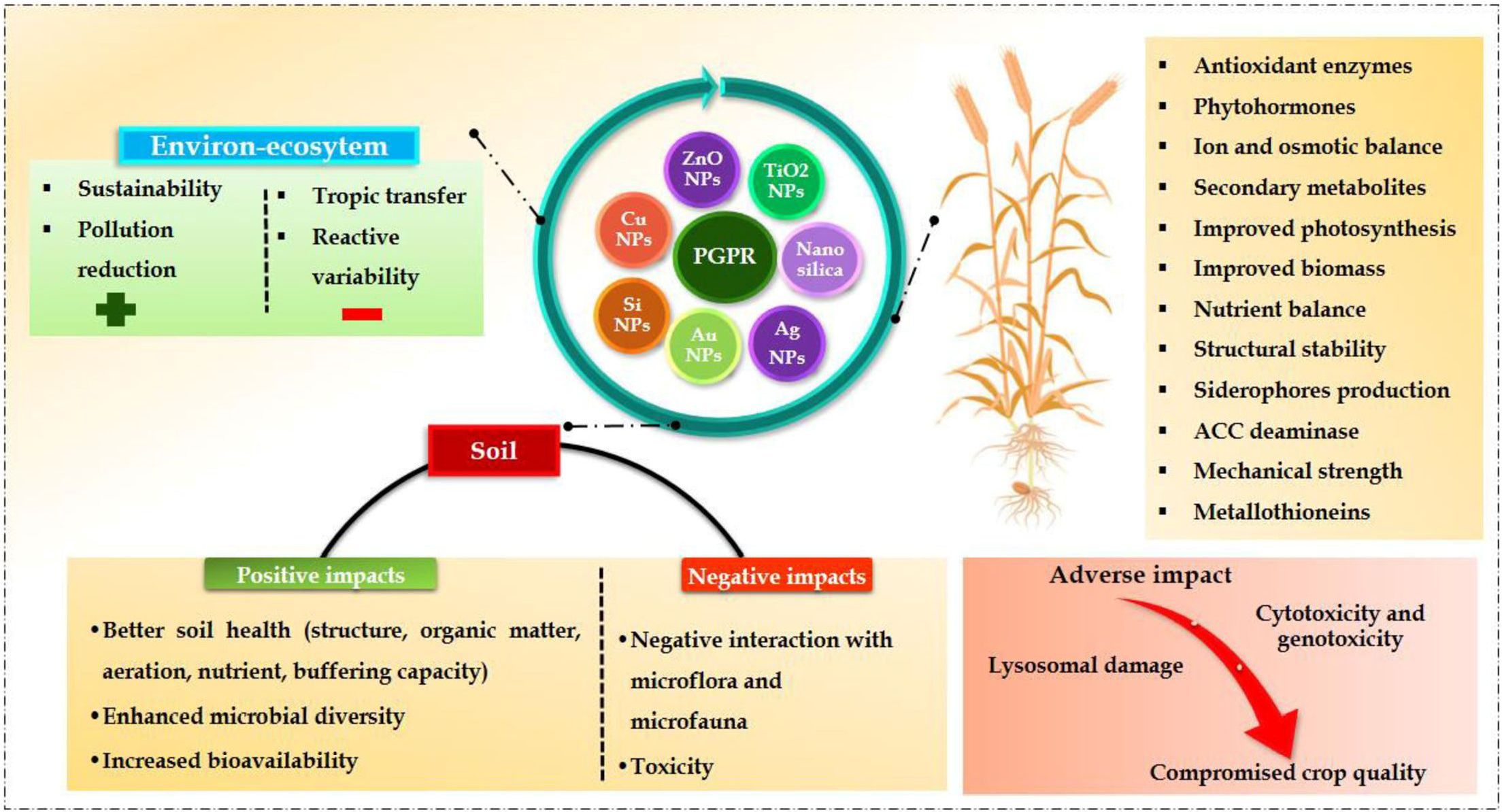
Figure 1 An overview of the interactive effects of NPs and PGPRs on plant, soil and enviro-ecosystems.
Sustainable food security and safety have become extremely challenging in the 21st century, particularly in developing nations with limited resources. The teeming millions in the developing world associated with the era of climate change threaten agricultural crop production and management, a serious challenge to sustainable agriculture (Fanzo et al., 2017; Watts et al., 2018). According to the Food and Agriculture Organization of the United Nations, over 2 billion people do not have enough food to eat due to the COVID-19 pandemic. Agriculture systems and food have already experienced significant modifications, but additional research has to be done in light of the transforming global landscape (Frona and Szenderák 2018; Kakaei et al., 2022). Land reforms, modified water management, stress-tolerant cultivars, increased fertilizer use, better seed, pesticide use, genetically modified crops, plant growth regulators, and soil amendments are some of the approaches used to improve soil quality and crop yields (Urra et al., 2019; Abdul Aziz et al., 2022; Verma et al., 2022c; Akanmu et al., 2023).
Crop yield downregulated because of various environmental stresses (Verma et al., 2020a; Kumari et al., 2022; Patni et al., 2022; Verma et al., 2023b). Nearly 6% of the world’s total surface area (1125 mha), impacted by salinity; it includes 20% of agricultural land and 33% of irrigated land. The loss of productivity from saline soil can exceed 46 mha annually (Hossain, 2019; Negacz et al., 2022). Annually, 1.5 mha of cropland is lost to saltwater due to soil erosion. Production of crops and animals requires more water as agriculture worldwide, provides 70% of total water returns. Water deficit is a major abiotic stressor prevalent in sub-tropical and tropical regions around the world (Kumari et al., 2022). Furthermore, droughts are becoming more severe due to the era of climate change. Water for agriculture has the potential to become more in requirement globally by 60% upto 2025. The development of plants and production is regularly reduced during drought stress because of insufficient nutrient availability, lower leaf photosynthetic CO2 assimilation rate and inadequate water availability (Figure 2). Moreover, dehydration increases plants’ biological ethylene synthesis, inhibiting the length and development of their roots (Hanjra and Qureshi, 2010; Mancosu et al., 2015; Boretti and Rosa, 2019; Verma et al., 2023b).
Heavy metals in soils are a major abiotic stressor that reduces agricultural output. Around the world, substantial amounts of heavy metals are frequently present in the soil because of several natural and human activities. Globally, over 10 million locations of polluted soil have been monitored, with a majority of 50% polluted areas with toxic ions. The toxic ions come into the agricultural land from different types of industries, coal burning, wastewater irrigation systems, petrochemical/hydrocarbon spillage, coal combustion, animal waste, and sewage sludge (Boretti and Rosa, 2019; Urra et al., 2019; Rashid et al., 2023; Verma et al., 2023b).
Currently, co-applying PGPRs and NPs has been used in several studies to enhance agronomic productivity and soil quality in different scenarios (Figures 1, 2). The explicit assumption in these studies has been that the NPs would make more nutrients available and generate a conducive habitat for the PGPRs to establish. In response, the latter would carry out their specific work (production of plant hormones, solubilization of nutrients, etc.) at maximum levels. Both stressed and non-stressed soils were used for these analyses. In the meantime, these demonstrations have yet to undergo an in-depth synthesis and critical evaluation. This review article aims to address the knowledge gap. Additionally, highlights the research avenues that will be explored soon to fully utilize the combined potential of PGPR and NPs for sustainable agroecosystems in the years to come.
Enhancing Soil Quality through Nanoparticle and PGPR Interactions
Because the soils carry out a wide range of ecological functions, it is characterized from the viewpoint of those functions. From the perspectives of concurrent agriculture and the environment, it is described as “the capacity of a soil to function within ecosystem and land-use boundaries to sustain biological productivity, maintain environmental quality, and promote plant and animal health” (Verma et al., 2022d; Anikwe and Ife, 2023; Sharma et al., 2023). The main chemical elements of soil quality are soil organic matter, pH, and accessible macronutrients (nitrogen, phosphorus, and potassium). Comparably, the most widely utilized biological indicators are soil respiration, microbial biomass, nitrogen mineralization, and extracellular enzymatic activities, whereas the most used physical indicators are bulk density, structural stability, and the retention of water (Nielsen and Winding, 2002; Bunemann et al., 2018). This review will evaluate the contribution of co-applying NPs and PGPR to enhance soil quality based on these parameters. Co-applying NPs and various PGPRs has been suggested as an effective approach to improving soil quality. Because NPs provide PGPR with a substrate with a high surface area and increased nutrition for their survival, their presence may enhance PGPR efficiency (Nayana et al., 2020; Akhtar et al., 2021; Alharbi et al., 2023; Rajput et al., 2023b; Verma et al., 2023b). The impact of co-applying different PGPRs and NPs on crop productivity and soil quality has been explored in the subsequent sections (Figures 1, 2; Table 1).
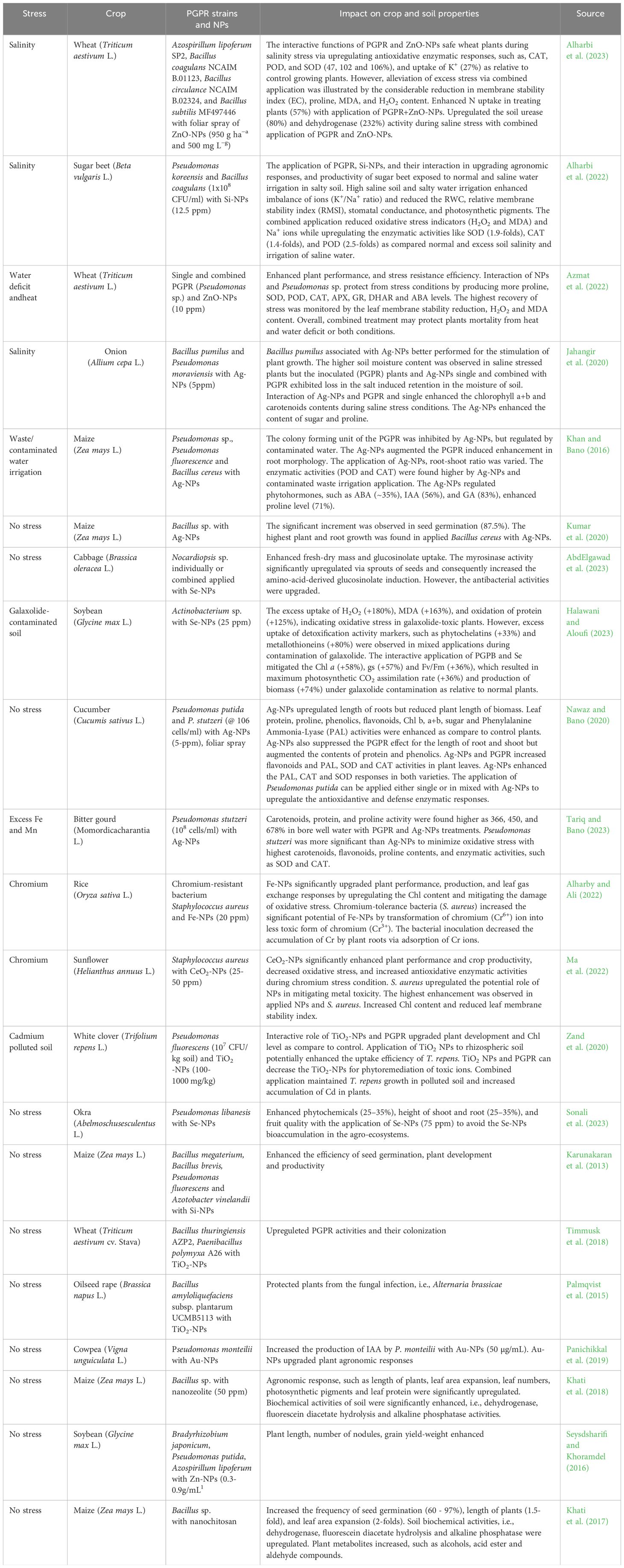
Table 1 Influence of co-applied PGPRs and nanoparticles on plants in response to normal and stressed conditions.
Nutrient Enhancement in Soil: The Role of Nanoparticles and PGPR
Numerous demonstrations assessed the impact of co-applying PGPR and NPs on soil quality, characterizing the physical, chemical, and biological characteristics of soil (Table 1). When NPs and PGPRs are applied together, generally found to enhance the level of mineral nutrient content in rhizospheric soils when compared to a single application of NPs or PGPR (Akhtar et al., 2021; Singh et al., 2021; Alharbi et al., 2023; Tang et al., 2023). The combined use of NPs and PGPRs resulted in increased soil nitrate levels compared to a single dose of nitrogen. However, PGPR and NPs enhanced organic carbon, phosphorus, and nitrogen availability compared to normal plants. Nanoparticles sprayed on plant leaves at low concentrations since they are economical and environmentally beneficial (Ameen et al., 2021; Liu et al., 2022; Verma et al., 2023a). NPs acquired more interest from plant physiologists because of their usage as nano-growth regulators to improve the growth and development of plants, mainly owing to the effective supply and accumulation and translocation of required minerals (Awan et al., 2020; Kupe et al., 2023). When mixed in soil, they dissolve nutrients due to dissolution and decomposition under the influence of soil properties and the activity of microbes. PGPR, especially those that solubilize organic phosphate, accelerates the accumulation of mineral nutrients from NPs. By utilizing PGPR and NPs together, soil microorganisms are spared from the need to seek out certain nutrients, enabling them to focus on the uptake of other essential elements. This shift enhances enzyme activity and facilitates the release of additional nutrients, thus promoting a more efficient nutrient acquisition process and overall soil fertility enhancement (Ameen et al., 2021; Liu et al., 2022; Rajput et al., 2023a).
Improving soil water retention with nanoparticles and PGPR
With their higher surface area-to-volume ratio, NPs can potentially increase soil water-holding capacity (WHC), especially those with coarse textures. The ameliorative impact of NPs on water retention capacity has also been found in multiple states of the experiments evaluating the co-application of NPs and PGPR. In comparison to PGPR and NPs application only, combined application of PGPR and NPs enhanced soil water holding capacity (WHC) (Nayana et al., 2020; Tang et al., 2023). While single use of PGPR has never been shown to improve soil WHC and water content, it can increase water-deficit resistance capacity to crop plants. However, enhanced WHC by the application of NPs, synergize with PGPR given that the nutrient cycling, breakdown of soil organic matter, and microbial signaling considering higher soil moisture levels [Figure 2] (Verma et al., 2020b; Ahmad et al., 2022; Chieb and Gachomo, 2023). It should be highlighted that the indirect significance of NPs with PGPR application has yet to be conducted.
Modulating soil microbial communities
Nanoparticles enhance various physicochemical characteristics of soil, eventually facilitating the function of indigenous soil microbial populations. NPs can boost WHC, soil pH, substrate, and nutrient availability, enhancing microbial biomass, abundance, and diversification (Rajput et al., 2023a). Furthermore, it has been demonstrated that NPs upregulate the nodulation of the natural rhizobia with legume plants. This is due to the enhancement in aeration provided by NPs that access more air to nodule bacteria, which can persist for a long time on the porous surface of NPs before colonization in the roots (Swarnalakshmi et al., 2020; Flores-Duarte et al., 2022).
The mutualistic relationship between existing microorganisms and plants may be further enhanced by adding NPs (Ameen et al., 2021). The phosphate-solubilizing bacteria Pseudomonas sp. increased the availability of phosphorus in soil presumably by solubilizing it from the NPs thereby increasing the colonization of roots and overall plant development (De Souza-Torres et al., 2021). The general abundance of some microbial groups in soil may increase with the interactive role of NPs and PGPR, which improves soil health throughout (Table 1). The authors attributed it increase in advantageous bacteria to the improved soil organic matter content and its breakdown due to the interacting effect of NPs and the inoculant (De Souza-Torres et al., 2021; Ahmad et al., 2022; Rajput et al., 2023b).
Catalyzing soil enzyme activity with nanoparticles and PGPR
Research studies on various intra- and extracellular enzymes have also been used to evaluate the potential benefits of applying NPs and PGPR simultaneously. Soil urease and dehydrogenase enzymes activity was enhanced by applying PGPR and NPs compared to single-use of any of them (Alharbi et al., 2023; Upadhayay et al., 2023). Jabborova et al. (2021) observed that co-inoculation of PGPRs with NPs resulted in higher levels of protease, alkaline, and acid phosphomonoesterase than a single application of PGPRs. Interactive application of Bacillus subtilis with NPs was significantly observed in the activities of invertase and catalase in soil than in single use of NPs (Medina-Velo et al., 2017; Khanna et al., 2021). Ultimately, it has been revealed that the PGPR with NPs increases the enzymatic activity of soil phosphomonoesterase in acidic and alkaline conditions, as well as that of sucrase, urease, protease, and invertase. By defining the direction and intensity of nutrient transformation processes in soil, these enzymes can improve soil fertility by stimulating biochemical processes within the ecosystem. There is a direct correlation between soil nutrients and the enhanced enzyme activity caused by PGPR and NPs (Mushtaq et al., 2020; Ahmad et al., 2022; Rajput et al., 2023b). Research is still lacking on how co-application of NPs and PGPRs affects leucine aminopeptidase and N-acetyl-glucosaminidase, significant N-cycling enzymes. These enzymes catalyze the complex proteinaceous compounds in soil. The amount of mineral nitrogen added to the NPs can be determined by measuring the activity of these enzymes in the presence of PGPR since the NPs are organic materials that contain organic proteins (Figures 1, 2; Table 2).
Nanoparticles and PGPR: a synergistic approach to combat environmental stresses
Crops that grow with low pesticide application concentrations, higher nutritional values, and disease resistance are necessary for sustainable agriculture. In recent decades, the widespread application of expensive agrochemicals in agriculture has prompted the development of more environmentally friendly substitutes, like PGPR and NPs (Medina-Velo et al., 2017; Ahmad et al., 2022). PGPR and NPs have been broadly mentioned for their significant impacts on plants. However, utilizing PGPR and NPs together has been more successful in plant production in recent years than using PGPR or NPs individually. Multiple research projects have documented the significant role of PGPR and NPs in enhancing crop productivity (Mushtaq et al., 2020; Khanna et al., 2021; Rajput et al., 2023a). Similarly, the combined use of Alcaligenes sp. with NPs promoted fresh and dry mass, plant length, yield, and quality of fruits over a single use of PGPR. PGPRs with NPs have also been evaluated during decreased fertilizer frequency to reduce the greenhouse gas emissions associated with the fabrication of ammoniac fertilizers and their volatilization (Awan et al., 2020; Mushtaq et al., 2020; Khanna et al., 2021; Shah et al., 2021).
Application of Enterobacter, Pseudomonas, Azospirillum, Agrobacterium and NPs enhanced sustainable agriculture production. The interactive use of PGPR and NPs may enhance seed germination frequency, height of plants, dry-fresh biomass, and crop productivity than the single use of PGPR or NPs (Medina-Velo et al., 2017; Fadiji et al., 2022; Sharma et al., 2023). This combination can work in different directions. In the direct mechanisms, the usual generation of plant hormones by the PGPR, such as indole acetic acid, siderophores, etc., and enhancing soil minerals’ availability through phosphate solubilization and N2 fixation contributes to better plant performance and productivity. The existence of NPs can assist the withstanding of the PGPR in larger numbers in addition to providing nutrient-rich substrate thereby leading to improved efficiency by the PGPR ultimately boosting the production of plants (Mushtaq et al., 2020; Khanna et al., 2021; Ahmad et al., 2022; Rani et al., 2023).
It has been widely recognized that the PGPR reducing a wide range of environmental stresses that impede the growth and development of plants. Many researchers have demonstrated their effectiveness in combating drought, soil flooding, salinity, low and high light intensities, nutritional imbalance, and heavy metal contamination. Plants can develop stress tolerance efficiency by exploiting the ability of PGPR to release exopolysaccharides in dry environments (Vurukonda et al., 2016; Ahmad et al., 2022; Chieb and Gachomo, 2023). In saline environments, it increases water absorption, decreases stomatal conductance, boosts potassium accumulation at the cost of sodium, reduces the direct negative impacts of soil salinity, and increases antioxidant enzyme activities. All these alterations assist in the improved growth of plants in saline environments. Similarly, it has been observed that the PGPR improves overall nutrient uptake while also immobilizing and reducing the uptake of heavy metals by plants, thereby reducing the toxicity caused by heavy metals (Bishnoi, 2014; Islam et al., 2014; Kaushal and Wani, 2016; Kumar et al., 2017; Etesami and Maheshwari, 2018). Bacillus pumilus in linked with Ag-NPs performed better for the stimulation of onion plant growth. Combined application exhibited a reduction in the salt-induced retention in the moisture of rhizospheric soil and enhanced protein content of bulb, reduced leaf flavonoids. Ag-NPs enhanced sugar and proline levels. Bacillus pumilus proved to be more significant during control conditions to all growth agronomic responses but Pseudomonas moraviensis potentially coped in response to saline conditions (Jahangir et al., 2020).
Numerous articles have reviewed these findings discussed in the Table 1. Additionally, it has been demonstrated that NPs improve salt tolerance, reduce drought stress, and minimize the toxicity that organic and inorganic soil contaminants cause in plants (Rasheed et al., 2022). Drought stress benefit in NPs-amended soils occurs through higher water holding capacity to large surface area-to-volume ratio of NPs (El-Saadony et al., 2022; Verma et al., 2022b, c; Verma et al., 2023a). Similarly, plants in soil amended with NPs mitigate soil saline conditions due to reduced osmotic stress to increased soil water content and decreased Na+ absorption caused by Na+ transient binding on sorption sites on NPs. The primary approach by which NPs reduce the toxicity stress of organic and inorganic heavy metals sorption. Multiple studies have reviewed all these applications of NPs against diverse environmental stressors (Azameti and Imoro, 2023; Faizan et al., 2023; Rajput et al., 2023b). Recently, some studies have explored the possibility of combining PGPR and NPs to mitigate the environmental circumstances for plant development with the assumption that both additives would act synergistically (Table 1). Synergies between PGPR and NPs have been actively explored in these studies.
Soil quality enhancement: the combined power of PGPR and nanoparticles
Improved soil quality occurs from the combined application of PGPR and NPs, which perform multiple functions to mitigate the effects of drought stress (Table 1). When the soil water content was half the field capacity (50% of soil moisture level), applying NPs and PGPR jointly substantially improved the pH, EC, nitrate, phosphorus, extractable K, and organic matter when compared by applying NPs and PGPR separately [Figures 1, 2] (Akhtar et al., 2021; Rajput et al., 2023a). Sun et al. (2022) reported that when the FeO-NPs were treated with arsenic (As)-contaminated soil with the PGPR P. vermicola, the outcomes were notably enhanced, yielding more effective results. Consequently, results demonstrated that the P. vermicola and FeO-NPs applied combined may mitigate As (arsenic) toxicity in seedlings of Trachyspermum ammi, improving plant growth and composition under metal stress observed by balanced exudation of organic acids (Sun et al., 2022). The developing direction from this research suggests that the higher water holding capacity and concurrent reduction in drought stress strengthen the survival and abundance of the PGPR, which perform their activities better (Ahmad et al., 2022).
In terms of soil quality, salt influences the composition of the microbial community in the soil and reduces biomass and microbial activity. Furthermore, under saline conditions, Na+ exceeds K+ transport channels, resulting in decreased and inhibited development of plants (Al-Turki et al., 2023). Despite this, it has been demonstrated that co-application of PGPR and NPs in salty conditions induces salt tolerance and plant development, mostly through lowering Na+ absorption and elevating the K+/Na+ ratio. Using two endophytic bacteria, such as Burkholderia phytofirmans or Enterobacter sp., and NPs drastically reduced saline stress in crop plants by minimizing the uptake of xylem Na+ content. On the contrary, combined application greatly enhanced the K+ and K+/Na+ ratio, reducing plant saline stress (Kumawat et al., 2022; Al-Turki et al., 2023; Rajput et al., 2023b). In the same demonstration, the sodium adsorption ratio and Na+ in soil solution were reduced by the latter application to adsorption sites and desorption of K+ by interactive combination. When inoculated with NPs and Paraburkholderia phytofirmans, which can produce exopolysaccharides—significantly reduced the level of Na+ in the soil solution, alleviating plant salinity stress (Fu and Yan, 2023).
Combined PGPRs and NPs have synergistic benefits on soil quality because they reduce the Na+ level and increase colonization efficiency. PGPR strains co-applied with NPs in salty soil showed higher colonizing efficacy than PGPRs without NPs in the soil (Kumawat et al., 2022; Rajput et al., 2023b). Enterobacter sp. with 5% NPs demonstrated increased colonizing efficiency in saline soil than Burkholderia phytofirmans with and without NPs. Compared to PGPR inoculation individually; co-applicating an endophytic PGPR with NPs produced in salty soil caused an increase in PGPR colonization in the rhizosphere, root, and shoot interior bacterial population of about 150–250%. In rhizospheric soil, NPs demonstrated downregulation in the Na+/K+ ratio and improved PGPR root colonization efficiency, reducing soil salinity stress. By releasing mineral elements like K+, Ca2+, and Mg2+ from the soil solution, NPs, and PGPRs maintain the nutritional balance by lowering the concentration of Na+ in the soil. As a result, the soil K+/Na+ ratio gradually improved. Na+ in soil binds by exopolysaccharides formed by PGPRs under stress (deMoraes et al., 2021; Kumawat et al., 2022; Fu and Yan, 2023). The specific genes responsive to stress in plants under the influence of NPs and PGPRs can widely depend on the type of stress, plant varieties/species, type of NPs, type of application, and applied PGPRs strains. Some of the stress-responsive genes in plants can be regulated by NPs and PGPRs which are shown in Table 2. It provides an overview of various gene categories that may be impacted by the presence of NPs and PGPRs in plants, along with their general functions in stress response.
In polluted soils, the application of PGPR together with NPs has also been explored (Table 1). Based on the findings; it is an effective approach for lowering soil contamination from heavy metals. Enterobacter sp. microbe with NPs could efficiently expedite the restoration of soil contaminated with cadmium (Cd) toxicity. The combined application of Bacillus sp. and NPs enhanced soil enzyme (dehydrogenase) more than NPs, leading to improved biological remediation. This combination also reduced HOAc-extractable Cd levels than independent applications of NPs and PGPR. By lowering the heavy metal’s availability, Bacillus sp. applied with NPs significantly reduced the detrimental impact of chromium and improved plant health. PGPRs and NPs immobilize metals via metal-immobilizing bacteria, adsorption, co-precipitation, and complexation, therefore restricting their availability in soil for uptake and translocation (Mitra et al., 2018; deMoraes et al., 2021; Zulfiqar et al., 2023).
Synergistic role of PGPR and nanoparticles enhancing physiological and yield characteristics
Several studies have demonstrated that the role of PGPR and NPs on plant performance in adverse agroclimatic conditions (Table 1). The impact of applying PGPR and NPs together on plant development and productivity has been reviewed, and different physiological and biochemical responses have been triggered (Rajput et al., 2023a). However, a consequence of drought stress is increased plant ethylene levels. It has been demonstrated that the use of ACC-producing deaminase-producing PGPR with NPs may reduce the higher ethylene level in plants caused by drought because the latter increases colonization in the plant rhizosphere and promotes the inoculant survival rate (deMoraes et al., 2021; Al-Turki et al., 2023). In terms of comparison, this resulted in higher plant yields than using PGPR or NPs alone. Comparably, using P. aeruginosa and NPs jointly decreased electrolyte leakage substantially compared to applying independently. Also, co-application improved fresh and dry leaf-shoot-root weight compared to a single usage of NPs or PGPR, according to deMoraes et al. (2021). When combined with NPs, several additional PGPRs that produce ACC deaminase, such as Agrobacterium fabrum and Bacillus amyloliquefaciens have also been shown to increase wheat productivity during severe water stress conditions [Table 1] (Al-Turki et al., 2023; Rajput et al., 2023a).
PGPR applied along with NPs to stressed plants, enhanced relative water content, stomatal conductance, Ca2+ and K+ levels, and reduced proline level. Additionally, researchers observed the reduced electrolyte leakage assisted plants in adapting to drought stress conditions. Drought causes plants to release more ethylene and electrolytes, which inhibits plant growth. By reducing ethylene concentration and electrolyte leakage in plants, co-application of PGPR with NPs may mitigate drought stress in plants. According to experiments carried out by Ahluwalia et al. (2021); Alharbi et al. (2023) and Chandrashekar et al. (2023), PGPR with NPs increased the relative water content, stomatal conductance, chlorophyll, and carotenoids in plants.
The salty soil influences plant development, growth, photosynthesis, lipid metabolism, and protein synthesis (Figures 1, 2). Hormonal imbalances and osmotic changes harm plant growth in saline soils (Singh et al., 2013). It also results in specific toxicity of ions and malnutrition. A different reason is that both sodium and chloride ions restrict plant growth. In certain plants, only chloride ions accumulate in the shoot, while sodium ions are retained in the roots and stems (Kumawat et al., 2022; Fu and Yan, 2023). When PGPRs and NPs are applied in common, their combined effects normally have a more beneficial impact on plant productivity and the elimination of salt stress than separate applications. Under salinity stress, combining NPs and a siderophore-producing strain of Burkholderia phytofirmans enhanced the plant height, grain yield, photosynthetic leaf gas exchange, and root and shoot dry weight, respectively. The synergistic ability to integrate PGPR and NPs to ameliorate soil salinity stress for plants has also been confirmed by evidence from multi-year field research experiments (deMoraes et al., 2021; Kumawat et al., 2022; Alharbi et al., 2023). PGPR and NPs play a major role in restoring toxic ions in plants. They can alter, accumulate or eliminate heavy metals (Gulzar and Mazumder, 2022; Wang et al., 2022; Rai et al., 2023).
When combined with NPs, Enterobacter sp. significantly facilitated the development of Brassica napus in cadmium-contaminated soil (Saeed et al., 2019). Compared to soil without PGPR and NPs under stress conditions, the co-application greatly enhanced shoot and root length, respectively. In addition, PGPR with NPs application reduced Cd uptake in root and shoot under Cd stress conditions relative to individual use of PGPR and NPs, respectively. An increment was observed in ryegrass biomass than the application of NPs, and minimum Cd level was noticed in the interactive application as relative to NPs, PGPR, and control (Jin et al., 2016; Rizwan et al., 2021).
Recent studies performed by Timmusk et al. (2018) underscore the transformative potential of integrating titania nanoparticles (TNs) with Plant Growth-Promoting Rhizobacteria (PGPR) in agriculture. This novel approach has demonstrated a significant boost in plant biomass, particularly under challenging conditions such as drought, salt, and pathogen stress. The synergy between TNs and PGPR not only fortifies plant resilience but also paves the way for sustainable yield improvements. By harnessing the combined power of these two agents, we can unlock new pathways to bolster plant health and productivity, marking a significant step forward in our quest for sustainable agricultural practices.
Deciphering the interactions: how PGPR and nanoparticles influence plant biology
When NPs are applied with PGPR strains, they provide a habitat for PGPR (i.e., colonization, reproduction, and growth) due to its porous structure, more surface area, and the capacity to absorb microorganisms and organic compounds. Several studies mentioned in Table 1 demonstrated that the adding NPs to soils increases the growth and abundance of PGPR inoculants. NPs also protected them from other dangerous bacterial infections. It provides energy and the essential nutritional building blocks for inoculants’ survival and growth. Furthermore, using NPs influences the physicochemical characteristics of soils and may increase soil microbial biomass and enzymatic activity. NPs are rich in different nutritional compositions, such as nitrogen, phosphorus, potassium, calcium, magnesium, zinc, etc., depending on the type and frequency of application (deMoraes et al., 2021; Singh et al., 2021; Alharbi et al., 2023; Al-Turki et al., 2023; Fu and Yan, 2023; Verma et al., 2023a).
PGPRs enhance plant growth through direct and indirect processes during normal and stressful situations. Like NPs, the PGPR can either bring in a nutrient from outside via their direct functions such as nitrogen fixation (by nitrogen-fixing bacteria) or solubilize the immobilized nutrients (by phosphate-solubilizing bacteria) thereby contributing to plant nutrition [Figures 1, 2, Table 1] (deMoraes et al., 2021). In addition, due to their nitrogen-fixing characteristics, nitrogen-fixing PGPRs like Paenibacillus polymyxa, Rahnella sp., and Serratia sp. can increase the level of mineral nitrogen in soil solution and restrict it from leaching into the soil (Weselowski et al., 2016; Liu et al., 2019; Ribeiro et al., 2020). A wide range of phosphate-solubilizing PGPRs has been demonstrated to solubilize and provide phosphate in soil for plant uptake (Walpola and Yoon, 2013; Alori et al., 2017; Prabhu et al., 2018; Prakash and Arora, 2019; Gupta et al., 2021; Koczorski et al., 2023; Suleimanova et al., 2023).
The direct phosphorus accumulation from NPs using PGPR has yet to be demonstrated. Similar mechanisms of higher availability of potassium may be predicted because PGPR is known for reducing the soil pH and making the soil potassium available to plants and NPs to be rich in essential minerals (deMoraes et al., 2021; Koczorski et al., 2023). Another direct pathway of the production of ACC deaminase reduces the generation of ethylene-enhanced levels obtained during stress conditions via its breakdown into ammonia and alpha ketobutyrate. Enterobacter sp., Alcaligenes sp., Pseudomonas fluorescens, Serratia odorifera, Leclerciaade carboxylata, Agrobacterium fabrum, Bacillus amyloliquefaciens, Pseudomonas aeruginosa, etc., can be produced by ACC deaminase. These strains have beneficial synergistic effects with NPs during environmental stress mitigation. PGPRs by their indirect processes, i.e., pH regulations, production of exopolysaccharides, and defense against biotic stresses are also associated with plant growth enhancement (Alori et al., 2017; Prakash and Arora, 2019; Singh et al., 2021; Kumawat et al., 2022; Alharbi et al., 2023; Suleimanova et al., 2023).
Potential drawbacks and risks associated with their use of NPs and PGPRs for sustainable crop development
Nanoparticles offer transformative potential for agriculture through their unique properties, such as high reactivity and the ability to be engineered for specific tasks. Still, they also present environmental and health risks. Concerns include the toxicity of nanoparticles to non-target organisms like soil microbes, plants, and animals, with studies indicating that certain nanoparticles can disrupt soil microbial communities and accumulate in the food chain. Their small size facilitates mobility, raising the risk of environmental contamination and unknown long-term effects (Tourinho et al., 2012; (Dimkpa et al., 2013; deMoraes et al., 2021). Human health concerns also arise from the potential for nanoparticles to enter the body through food consumption, with evidence of their ability to cross biological barriers. Regulatory challenges are significant, as existing frameworks may not adequately address the risks associated with nanomaterials. Despite these risks, the benefits of nanoparticle use in agriculture are considerable, necessitating comprehensive risk assessments, safe handling practices, and clear regulatory guidelines to ensure their safe and sustainable application ( (Grieger et al., 2012; Shah et al., 2021). To ensure a sustainable approach, it is crucial to conduct comprehensive risk assessments, invest in research for understanding long-term effects, and develop nanomaterials that are biodegradable and safe for the environment and human health. Furthermore, establishing clear regulatory guidelines and promoting safe handling practices are essential for mitigating risks (Grieger et al., 2012). By prioritizing safety and sustainability, the agricultural sector can leverage nanotechnology to address global food security challenges while protecting environmental health and biodiversity.
Conclusions and future perspectives
During adverse agroclimatic conditions, reduced plant growth and crop mortality are prevalent through significant food and commercial cash crops. Given the significant potential of PGPR and NPs to enhance crop productivity and soil health under both normal and adverse conditions, our conclusion highlights the necessity of integrating these technologies into sustainable agricultural practices. The synergy between PGPR and NPs not only offers a path to reducing synthetic fertilizer dependency but also promises resilience against climatic stresses by improving plant performance, production, and fruit or grain quality, as well as soil profile. However, as highlighted in this article, limited field demonstrations have been conducted to assess the significance of the PGPR and NPs’ interactive role in sustainable agriculture. Mechanistic research on the interaction between PGPR and NPs requires more research. An extremely efficient approach to evaluate the combined impact of PGPR and NPs could be long-duration field demonstrations. The potential of the PGPR and NPs for sustainable food production has been independently assessed in reasonably long-duration field studies.
Future research is poised to delve deeper into the mechanistic underpinnings of PGPR and NP interactions, with a strong emphasis on long-term field trials. These studies are critical for understanding the dynamic interactions in various soil types, especially those lacking in organic matter in tropical and subtropical regions. The aim is to develop tailored application strategies that leverage the unique benefits of PGPR and NPs, thereby reducing reliance on synthetic fertilizers and enhancing the environmental sustainability of agricultural systems. This detailed exploration and application of PGPR and NPs hold the promise of revolutionizing farming practices to meet the global food demand sustainably.
Author contributions
KV: Writing – original draft, Resources, Methodology, Formal analysis, Data curation, Conceptualization. AJ: Writing – original draft, Software, Resources, Formal analysis, Data curation. X-PS: Writing – review & editing, Visualization, Validation, Supervision, Project administration, Methodology, Investigation, Funding acquisition, Conceptualization. SS: Writing – review & editing, Software, Resources, Data curation. AK: Writing – review & editing, Software, Resources, Formal analysis, Data curation. JA: Writing – review & editing, Software, Resources, Formal analysis, Data curation. SKS: Writing – review & editing, Software, Resources, Formal analysis, Data curation. MS: Writing – review & editing, Software, Resources, Formal analysis, Data curation. CS: Writing – review & editing, Software, Resources, Formal analysis, Data curation. Y-RL: Writing – review & editing, Visualization, Validation, Supervision, Software, Project administration, Methodology, Investigation, Funding acquisition, Conceptualization.
Funding
The author(s) declare financial support was received for the research, authorship, and/or publication of this article. This research was financially supported by the Guangxi Natural Science Foundation (2021GXNSFAA220022), Guangxi Innovation Teams of Modern Agriculture Technology (nycytxgxcxtd-2021-03), Guangxi Characteristic Crop Experimental Station (GTS2022022), National Key Research and Development Project (2022YFD2301102-07), The National Natural Science Foundation of China (31760415), Fund of Guangxi Academy of Agricultural Sciences (2021YT011) and Science and Technology Major Project of Guangxi (Guike AA22117002-1).
Acknowledgments
The authors would like to thank the Guangxi Academy of Agricultural Sciences, Nanning, Guangxi, China, for providing the necessary facilities for this study.
Conflict of interest
The authors declare that the research was conducted in the absence of any commercial or financial relationships that could be construed as a potential conflict of interest.
Publisher’s note
All claims expressed in this article are solely those of the authors and do not necessarily represent those of their affiliated organizations, or those of the publisher, the editors and the reviewers. Any product that may be evaluated in this article, or claim that may be made by its manufacturer, is not guaranteed or endorsed by the publisher.
References
AbdElgawad, H., Korany, S. M., Reyad, A. M., Zahid, I., Akhter, N., Alsherif, E., et al. (2023). Synergistic impacts of plant-growth-promoting bacteria and selenium nanoparticles on improving the nutritional value and biological activities of three cultivars of Brassica sprouts. ACS Omega 8, 26414–26424. doi: 10.1021/acsomega.3c02957
Abdul Aziz, M., Brini, F., Rouached, H., Masmoudi, K. (2022). Genetically engineered crops for sustainably enhanced food production systems. Front. Plant Sci. 13. doi: 10.3389/fpls.2022.1027828
Ahluwalia, O., Singh, P. C., Bhatia, R. (2021). A review on drought stress in plants: Implications, mitigation and the role of plant growth promoting rhizobacteria. Resour. Environ. Sust. 5, 100032. doi: 10.1016/j.resenv.2021.100032
Ahmad, H. M., Fiaz, S., Hafeez, S., Zahra, S., Shah, A. N., Gul, B., et al. (2022). Plant growth-promoting rhizobacteria eliminate the effect of drought stress in plants: A review. Front. Plant Sci. 13. doi: 10.3389/fpls.2022.875774
Akanmu, A. O., Akol, A. M., Ndolo, D. O., Kutu, F. R., Babalola, O. O. (2023). Agroecological techniques: Adoption of safe and sustainable agricultural practices among the smallholder farmers in Africa. Front. Sust. Food Syst. 7. doi: 10.3389/fsufs.2023.1143061
Akhtar, N., Ilyas, N., Mashwani, Z., Hayat, R., Yasmin, H., Noureldeen, A., et al. (2021). The synergistic effects of plant growth promoting rhizobacteria and silicon dioxide nano-particles to ameliorate drought stress in wheat. Plant Physiol. Biochem. 166, 160–176. doi: 10.1016/j.plaphy.2021.05.039
Alharbi, K., Hafez, E., Omara, A. E.-D., Awadalla, A., Nehela, Y. (2022). Plant growth promoting rhizobacteria and silica nanoparticles stimulate sugar beet resilience to irrigation with saline water in salt-affected soils. Plants 11, 3117. doi: 10.3390/plants11223117
Alharbi, K., Hafez, E. M., Omara, A. E. D., Rashwan, E., Alshaal, T. (2023). Zinc oxide nanoparticles and PGPR strengthen salinity tolerance and productivity of wheat irrigated with saline water in sodic-saline soil. Plant Soil 493, 475–495. doi: 10.1007/s11104-023-06245-7
Alharby, H. F., Ali, S. (2022). Combined role of Fe nanoparticles (Fe NPs) and Staphylococcus aureus L. @ in alleviating chromium stress in rice plants. Life (Basel) 12, 338. doi: 10.3390/life12030338
Ali, E. F., El-Shehawi, A. M., Ibrahim, O. H. M., Abdul-Hafeez, E. Y., Moussa, M. M., Hassan, F. A. S. (2021). A vital role of chitosan nanoparticles in improvisation the drought stress tolerance in Catharanthus roseus (L.) through biochemical and gene expression modulation. Plant Physiol. Biochem. 161, 166–175. doi: 10.1016/j.plaphy.2021.02.008
Alori, E. T., Glick, B. R., Babalola, O. O. (2017). Microbial phosphorus solubilization and its potential for use in sustainable agriculture. Front. Microbiol. 8. doi: 10.3389/fmicb.2017.00971
Al-Turki, A., Murali, M., Omar, A. F., Rehan, M., Sayyed, R. Z. (2023). Recent advances in PGPR-mediated resilience toward interactive effects of drought and salt stress in plants. Front. Microbiol. 14. doi: 10.3389/fmicb.2023.1214845
Ameen, F., Alsamhary, K., Alabdullatif, J. A., ALNadhari, S. (2021). A review on metal-based nanoparticles and their toxicity to beneficial soil bacteria and fungi. Ecotoxicol. Environ. Saf. 213, 112027. doi: 10.1016/j.ecoenv.2021.112027
Anikwe, M. A., Ife, K. (2023). The role of soil ecosystem services in the circular bioeconomy. Front. Soil Sci. 3. doi: 10.3389/fsoil.2023.1209100
Awan, S., Shahzadi, K., Javad, S., Tariq, A., Ahmad, A., Ilyas, S. (2020). A preliminary study of influence of zinc oxide nanoparticles on growth parameters of Brassica oleracea var italic. J. Saudi Soc Agric. Sci. 20, 18–24. doi: 10.1016/j.jssas.2020.10.003
Azameti, M. K., Imoro, A. M. (2023). Nanotechnology: A promising field in enhancing abiotic stress tolerance in plants. Crop Design 2, 100037. doi: 10.1016/j.cropd.2023.100037
Azmat, A., Tanveer, Y., Yasmin, H., Hassan, M. N., Shahzad, A., Reddy, M., et al. (2022). Coactive role of zinc oxide nanoparticles and plant growth promoting rhizobacteria for mitigation of synchronized effects of heat and drought stress in wheat plants. Chemosphere 297, 133982. doi: 10.1016/j.chemosphere.2022.133982
Bishnoi, U. (2014). PGPR interaction: An ecofriendly approach promoting the sustainable agriculture system. Adv. Bot. Res. 75, 81–113. doi: 10.1016/bs.abr.2015.09.006
Boretti, A., Rosa, L. (2019). Reassessing the projections of the world water development report. NPJ Clean Water 2, 1–6. doi: 10.1038/s41545-019-0039-9
Bunemann, E. K., Bongiorno, G., Bai, Z., Creamer, R. E., De Deyn, G., De Goede, R., et al. (2018). Soil quality – A critical review. Soil Biol. Biochem. 120, 105–125. doi: 10.1016/j.soilbio.2018.01.030
Cai, L., Cai, L., Jia, H., Liu, C., Wang, D., Sun, X. (2020). Foliar exposure of Fe3O4 nanoparticles on Nicotiana benthamiana: evidence for nanoparticles uptake, plant growth promoter and defense response elicitor against plant virus. J. Hazard. Mater. 393, 122415. doi: 10.1016/j.jhazmat.2020.122415
Chandrashekar, H. K., Singh, G., Kaniyassery, A., Thorat, S. A., Nayak, R., Murali, T. S., et al. (2023). Nanoparticle-mediated amelioration of drought stress in plants: A systematic review. 3 Biotech. 13, 336. doi: 10.1007/s13205-023-03751-4
Chen, C., Unrine, J. M., Hu, Y., Guo, L., Tsyusko, O. V., Fan, Z., et al. (2021). Responses of soil bacteria and fungal communities to pristine and sulfidized zinc oxide nanoparticles relative to Zn ions. J. Hazard. Mater. 405, 124258. doi: 10.1016/j.jhazmat.2020.124258
Chieb, M., Gachomo, E. W. (2023). The role of plant growth promoting rhizobacteria in plant drought stress responses. BMC Plant Biol. 23, 407. doi: 10.1186/s12870-023-04403-8
deMoraes, A. C. P., Ribeiro, L., deCamargo, E. R., Lacava, P. T. (2021). The potential of nanomaterials associated with plant growth-promoting bacteria in agriculture. 3 Biotech. 11, 318. doi: 10.1007/s13205-021-02870-0
De Souza-Torres, A., Govea-Alcaide, E., Gómez-Padilla, E., Masunaga, S. H., Effenberger, F. B., Rossi, L. M., et al. (2021). Fe3O4 nanoparticles and Rhizobium inoculation enhance nodulation, nitrogen fixation and growth of common bean plants grown in soil. Rhizosphere 17, 100275. doi: 10.1016/j.rhisph.2020.100275
Dilnawaz, F., Misra, A. N., Apostolova, E. (2023). Involvement of nanoparticles in mitigating plant’s abiotic stress. Plant Stress 10, 100280. doi: 10.1016/j.stress.2023.100280
Dimkpa, C. O., Latta, D. E., McLean, J. E., Britt, D. W., Boyanov, M. I., Anderson, A. J. (2013). Fate of CuO and ZnO nano-and microparticles in the plant environment. Environ. Sci. Technol. 47, 4734–4742. doi: 10.1021/es304736y
Elmer, W., de la Torre-Roche, R., Pagano, L., Majumdar, S., Zuverza-Mena, N., Dimkpa, C., et al. (2018). Effect of metalloid and metal oxide nanoparticles on Fusarium wilt of watermelon. Plant Dis. 102, 1394–1401. doi: 10.1094/PDIS-10-17-1621-RE
El-Ramady, H., Brevik, E. C., Fawzy, Z. F., Elsakhawy, T., Omara, A. E.-D., Amer, M., et al. (2022). Nano-restoration for sustaining soil fertility: A pictorial and diagrammatic review article. Plants 11, 2392. doi: 10.3390/plants11182392
El-Saadony, M. T., Saad, A. M., Soliman, S. M., Salem, H. M., Desoky, E., Babalghith, A. O., et al. (2022). Role of nanoparticles in enhancing crop tolerance to abiotic stress: A comprehensive review. Front. Plant Sci. 13. doi: 10.3389/fpls.2022.946717
Etesami, H., Maheshwari, D. K. (2018). Use of plant growth promoting rhizobacteria (PGPRs) with multiple plant growth promoting traits in stress agriculture: Action mechanisms and future prospects. Ecotoxicol. Environ. Saf. 156, 225–246. doi: 10.1016/j.ecoenv.2018.03.013
Fadiji, A. E., Orozco-Mosqueda, C., Santoyo, G., Babalola, O. O. (2022). Recent developments in the application of plant growth-promoting drought adaptive rhizobacteria for drought mitigation. Plants 11 (22), 3090. doi: 10.3390/plants11223090
Faizan, M., Alam, P., Rajput, V. D., Faraz, A., Afzal, S., Ahmed, S. M., et al. (2023). Nanoparticle mediated plant tolerance to heavy metal stress: what we know? Sustainability 15, 1446. doi: 10.3390/su15021446
Fanzo, J., McLaren, R., Davis, C., Choufani, J. (2017). Climate change and variability: What are the risks for nutrition, diets, and food systems? (Washington, USA: International Food Policy Research Institute IFPRI), 1–128.
Flores-Duarte, N. J., Mateos-Naranjo, E., Redondo-Gómez, S., Pajuelo, E., Rodriguez-Llorente, I. D., Navarro-Torre, S. (2022). Role of nodulation-enhancing rhizobacteria in the promotion of Medicago sativa development in nutrient-poor soils. Plants 11 (9), 1164. doi: 10.3390/plants11091164
Frona, D., Szenderák, J. (2018). The challenge of feeding the world. Sustainability 11, 5816. doi: 10.3390/su11205816
Fu, B., Yan, Q. (2023). Exopolysaccharide is required for motility, stress tolerance, and plant colonization by the endophytic bacterium Paraburkholderia phytofirmans PsJN. Front. Microbiol. 14. doi: 10.3389/fmicb.2023.1218653
Garbowski, T., Bar-Michalczyk, D., Charazińska, S., Grabowska-Polanowska, B., Kowalczyk, A., Lochyński, P. (2022). An overview of natural soil amendments in agriculture. Soil Tillage Res. 225, 105462. doi: 10.1016/j.still.2022.105462
Grieger, K. D., Linkov, I., Hansen, S. F., Baun, A. (2012). Environmental risk analysis for nanomaterials: review and evaluation of frameworks. Nanotoxicol 6, 196–212. doi: 10.3109/17435390.2011.569095
Gulzar, A. B. M., Mazumder, P. B. (2022). Helping plants to deal with heavy metal stress: the role of nanotechnology and plant growth promoting rhizobacteria in the process of phytoremediation. Environ. Sci. pollut. Res. Int. 29, 40319–40341. doi: 10.1007/s11356-022-19756-0
Gupta, R., Noureldeen, A., Darwish, H. (2021). Rhizosphere mediated growth enhancement using phosphate solubilizing rhizobacteria and their tri-calcium phosphate solubilization activity under pot culture assays in Rice (Oryza sativa.). Saudi J. Biol. Sci. 28, 3692–3700. doi: 10.1016/j.sjbs.2021.05.052
Halawani, R. F., Aloufi, F. A. (2023). Galaxolide-contaminated soil and tolerance strategies in soybean plants using biofertilization and selenium nanoparticle supplementation. Front. Plant Sci. 14. doi: 10.3389/fpls.2023.1221780
Hanjra, M. A., Qureshi, M. E. (2010). Global water crisis and future food security in an era of climate change. Food Policy 35, 365–377. doi: 10.1016/j.foodpol.2010.05.006
Hezaveh, T. A., Pourakbar, L., Rahmani, F., Alipour, H. (2019). Interactive effects of salinity and ZnO nanoparticles on physiological and molecular parameters of rapeseed (Brassica napus L.). Commun. Soil Sci. Plant Anal. 50, 698–715. doi: 10.1080/00103624.2019.1589481
Islam, F., Yasmeen, T., Ali, Q., Ali, S., Arif, M. S., Hussain, S., et al. (2014). Influence of Pseudomonas aeruginosa as PGPR on oxidative stress tolerance in wheat under Zn stress. Ecotoxicol. Environ. Saf. 104, 285–293. doi: 10.1016/j.ecoenv.2014.03.008
Jabborova, D., KanNepalli, A., Davranov, K., Narimanov, A., Enakiev, Y., Syed, A., et al. (2021). Co-inoculation of rhizobacteria promotes growth, yield, and nutrient contents in soybean and improves soil enzymes and nutrients under drought conditions. Sci. Rep. 11 (1), 1-9. doi: 10.1038/s41598-021-01337-9
Jahangir, S., Javed, K., Bano, A. (2020). Nanoparticles and plant growth promoting rhizobacteria (PGPR) modulate the physiology of onion plant under salt stress. Pak. J. Bot. 52, 1473–1480. doi: 10.30848/PAK.J.BOT
Jin, Y., Liu, W., Li, X., Shen, S., Liang, S., Liu, C., et al. (2016). Nano-hydroxyapatite immobilized lead and enhanced plant growth of ryegrass in a contaminated soil. Ecol. Engg. 95, 25–29. doi: 10.1016/j.ecoleng.2016.06.071
Kakaei, H., Nourmoradi, H., Bakhtiyari, S., Jalilian, M., Mirzaei, A. (2022). Effect of COVID-19 on food security, hunger, and food crisis. COVID-19 Sustain. Dev. Goals 3-29. doi: 10.1016/B978-0-323-91307-2.00005-5
Karunakaran, G., Suriyaprabha, R., Manivasakan, P., Yuvakkumar, R., Rajendran, V., Prabu, P., et al. (2013). Effect of nanosilica and silicon sources on plant growth promoting rhizobacteria, soil nutrients and maize seed germination. IET Nanobiotechnol. 7, 70–77. doi: 10.1049/iet-nbt.2012.0048
Kaushal, M., Wani, S. P. (2016). Rhizobacterial-plant interactions: Strategies ensuring plant growth promotion under drought and salinity stress. Agricul. Ecosyst. Environ. 231, 68–78. doi: 10.1016/j.agee.2016.06.031
Khan, N., Bano, A. (2016). Role of plant growth promoting rhizobacteria and Ag-nano particle in the bioremediation of heavy metals and maize growth under municipal wastewater irrigation. Int. J. Phytoremed. 18, 211–221. doi: 10.1080/15226514.2015.1064352
Khanna, K., Kohli, S. K., Handa, N., Kaur, H., Ohri, P., Bhardwaj, R., et al. (2021). Enthralling the impact of engineered nanoparticles on soil microbiome: A concentric approach towards environmental risks and cogitation. Ecotoxicol. Environ. Saf. 222, 112459. doi: 10.1016/j.ecoenv.2021.112459
Khati, P., Chaudhary, P., GAngola, S., Bhatt, P., Sharma, A. (2017). Nanochitosan supports growth of Zea mays and also maintains soil health following growth. 3 Biotech. 7, 81. doi: 10.1007/s13205-017-0668-y
Khati, P., Parul, Bhatt, P., Nisha, Kumar, R., Sharme, A. (2018). Effect of nanozeolite and plant growth promoting rhizobacteria on maize. 3 Biotech. 8, 141. doi: 10.1007/s13205-018-1142-1
Koczorski, P., Furtado, B. U., Baum, C., Weih, M., Ingvarsson, P., Hulisz, P., et al. (2023). Large effect of phosphate-solubilizing bacteria on the growth and gene expression of Salix spp. At low phosphorus levels. Front. Plant Sci. 14. doi: 10.3389/fpls.2023.1218617
Kumar, P., Pahal, V., Gupta, A., Vadhan, R., Chandra, H., Dubey, R. C. (2020). Effect of silver nanoparticles and Bacillus cereus LPR2 on the growth of Zea mays. Sci. Rep. 10, 20409. doi: 10.1038/s41598-020-77460-w
Kumar, A., Singh, V. K., Tripathi, V., Singh, P. P., Singh, A. K. (2017). Plant growth-promoting rhizobacteria (PGPR): perspective in agriculture under biotic and abiotic stress. Crop Improve. Through Microbial. Biotechnol., 333–342. doi: 10.1016/B978-0-444-63987-5.00016-5
Kumari, A., Dash, M., Singh, S. K., Jagadesh, M., Mathpal, B., Mishra, P. K., et al. (2023a). Soil microbes: a natural solution for mitigating the impact of climate change. Environ. Monit. Assess. 195, 1436. doi: 10.1007/s10661-023-11988-y
Kumari, A., Lakshmi, G. A., Krishna, G. K., Patni, B., Prakash, S., Bhattacharyya, M., et al. (2022). Climate change and its impact on crops: A comprehensive investigation for sustainable agriculture. Agronomy 12, 3008. doi: 10.3390/agronomy12123008
Kumari, A., Singh, S. K., Al-Tawaha, A. R. M., Thangadurai, D., Panigatti, S., Sangeetha, J. (2024). Effect of mycorrhizal inoculation on citrus seedling growth and nutrient uptake. In: Microbial fertilizer technology for sustainable crop production. Eds. Sangeetha, J., Al Tawaha, A. R. M., Thangadurai, D. (In-press), (Burlington, Canada: Apple Academic Press Inc.).
Kumari, A., Singh, S. K., Mathpal, B., Verma, K. K., Garg, V. K., Bhattacharyya, M., et al. (2023b). The biosynthesis, mechanism of action, and physiological functions of melatonin in horticultural plants: A review. Horticulturae 9, 913. doi: 10.3390/horticulturae9080913
Kumawat, K. C., Sharma, B., Nagpal, S., Kumar, A., Tiwari, S., Nair, R. M. (2022). Plant growth-promoting rhizobacteria: Salt stress alleviators to improve crop productivity for sustainable agriculture development. Front. Plant Sci. 13. doi: 10.3389/fpls.2022.1101862
Kupe, M., Hacimuftuoglu, F., Yağanoğlu, E. (2023). Effects of PGPR bacteria applications on soil properties, plant growth and yield values in Karaerik and Narince grape varieties. J. Agric. Prod. 4, 128–137. doi: 10.56430/japro.1372396
Liu, X., Li, Q., Li, Y., Guan, G., Chen, S. (2019). Paenibacillus strains with nitrogen fixation and multiple beneficial properties for promoting plant growth. PeerJ 7, e7445. doi: 10.7717/peerj.7445
Liu, L., Nian, H., Lian, T. (2022). Plants and rhizospheric environment: affected by zinc oxide nanoparticles (ZnO NPs). A review. Plant Physiol. Biochem. 185, 91–100. doi: 10.1016/j.plaphy.2022.05.032
Ma, J., Alshaya, H., Okla, M. K., Alwasel, Y. A., Chen, F., Adrees, M., et al. (2022). Application of Cerium dioxide nanoparticles and chromium-resistant bacteria reduced chromium toxicity in sunflower plants. Front. Plant Sci. 13. doi: 10.3389/fpls.2022.876119
Magnabosco, P., Masi, A., Shukla, R., Bansal, V., Carletti, P. (2023). Advancing the impact of plant biostimulants to sustainable agriculture through nanotechnologies. Chem. Biol. Technol. Agric. 10, 1–25. doi: 10.1186/s40538-023-00491-8
Mahakham, W., Sarmah, A. K., Maensiri, S., Theerakulpisut, P. (2017). Nanopriming technology for enhancing germination and starch metabolism of aged rice seeds using phytosynthesized silver nanoparticles. Sci. Rep. 7, 8263. doi: 10.1038/s41598-017-08669-5
Mancosu, N., Snyder, R. L., Kyriakakis, G., Spano, D. (2015). Water scarcity and future challenges for food production. Water 7, 975–992. doi: 10.3390/w7030975
Medina-Velo, I. A., Barrios, A. C., Zuverza-Mena, N., Hernandez-Viezcas, J. A., Chang, C. H., Ji, Z., et al. (2017). Comparison of the effects of commercial coated and uncoated ZnO nanomaterials and Zn compounds in kidney bean (Phaseolus vulgaris) plants. J. Hazard. Mater. 332, 214–222. doi: 10.1016/j.jhazmat.2017.03.008
Mitra, S., Pramanik, K., Sarkar, A., Ghosh, P. K., Soren, T., Maiti, T. K. (2018). Bioaccumulation of cadmium by Enterobacter sp. and enhancement of rice seedling growth under cadmium stress. Ecotoxicol. Environ. Saf. 156, 183–196. doi: 10.1016/j.ecoenv.2018.03.001
Mohamed, N. G., Abdel-Hakeem, M. A. (2023). Chitosan nanoparticles enhance drought tolerance in tomatoes (Solanum lycopersicum L) via gene expression modulation. Plant Gene 34, 100406. doi: 10.1016/j.plgene.2023.100406
Moradi, P., Vafaee, Y., Mozafari, A. A., Tahir, N. A.-R. (2022). Silicon nanoparticles and methyl jasmonate improve physiological response and increase expression of stress-related genes in strawberry cv. Paros under salinity stress. Silicon 14, 10559–10569. doi: 10.1007/s12633-022-01791-8
Mushtaq, T., Shah, A. A., Akram, W., Yasin, N. A. (2020). Synergistic ameliorative effect of iron oxide nanoparticles and Bacillus subtilis S4 against arsenic toxicity in Cucurbita moschata: polyamines, antioxidants, and physiochemical studies. Int. J. Phytoremed. 13, 1408–1419. doi: 10.1080/15226514.2020.1781052
Nawaz, S., Bano, A. (2020). Effects of PGPR (Pseudomonas sp.) and Ag-nanoparticles on enzymatic activity and physiology of cucumber. Recent Pat. Food Nutr. Agric. 11, 124–136. doi: 10.2174/2212798410666190716162340
Nayana, A. R., Joseph, B. J., Jose, A., Radhakrishnan, E. K. (2020). “Nanotechnological Advances with PGPR Applications,” in Sustainable Agriculture Reviews, vol. 41 . Eds. Hayat, S., Pichtel, J., Faizan, M., Fariduddin, Q. (Springer, Cham). doi: 10.1007/978-3-030-33996-8_9
Negacz, K., Malek, Z., De Vos, A., Vellinga, P. (2022). Saline soils worldwide: Identifying the most promising areas for saline agriculture. J. Arid Environ. 203, 104775. doi: 10.1016/j.jaridenv.2022.104775
Nielsen, M. N., Winding, A. (2002). Microorganisms as Indicators of Soil Health (Denmark: National Environmental Research Institute). Technical Report No. 388.
Oleńska, E., Małek, W., Wójcik, M., Swiecicka, I., Thijs, S., Vangronsveld, J. (2020). Beneficial features of plant growth-promoting rhizobacteria for improving plant growth and health in challenging conditions: A methodical review. Sci. Total Environ. 743, 140682. doi: 10.1016/j.scitotenv.2020.140682
Palmqvist, N. G. M., Bejai, S., Seisenbaeva, G. A., Kessler, V. G. (2015). Nano titania aided clustering and adhesion of beneficial bacteria to plant roots to enhance crop growth and stress management. Sci. Rep. 5, 10146. doi: 10.1038/srep10146
Panichikkal, J., Thomas, R., John, J. C. (2019). Biogenic gold nanoparticle supplementation to plant beneficial pseudomonas monteilii was found to enhance its plant probiotic effect. Curr. Microbiol. 76, 503–509. doi: 10.1007/s00284-019-01649-0
Patni, B., Bhattacharyya, M., Kumari, A., Purohit, V. K. (2022). Alarming influence of climate change and compromising quality of medicinal plants. Plant Physiol. Rep. 27, 1–10. doi: 10.1007/s40502-021-00616-x
Prabhu, N., Borkar, S., Garg, S. (2018). Phosphate solubilization by microorganisms: Overview, mechanisms, applications and advances. Adv. Biol. Sci. Res., 161–176. doi: 10.1016/B978-0-12-817497-5.00011-2
Prakash, J., Arora, N. K. (2019). Phosphate-solubilizing Bacillus sp. Enhances growth, phosphorus uptake and oil yield of Mentha arvensis L. 3 Biotech. 9, 126. doi: 10.1007/s13205-019-1660-5
Pramanik, B., Sar, P., Bharti, R., Gupta, R. K., Purkayastha, S., Sinha, S., et al. (2023). Multifactorial role of nanoparticles in alleviating environmental stresses for sustainable crop production and protection. Plant Physiol. Biochem. 201, 107831. doi: 10.1016/j.plaphy.2023.107831
Raeisi Sadati, S. Y., Jahanbakhsh Godehkahriz, S., Ebadi, A., Sedghi, M. (2022). Zinc oxide nanoparticles enhance drought tolerance in wheat via physio-biochemical changes and stress genes expression. Iran. J. Biotechnol. 20, e3027. doi: 10.30498/ijb.2021.280711.3027
Rai, P. K., Song, H., Kim, K. (2023). Nanoparticles modulate heavy-metal and arsenic stress in food crops: Hormesis for food security/safety and public health. Sci. Total Environ. 902, 166064. doi: 10.1016/j.scitotenv.2023.166064
Rajput, V. D., Kumari, A., Upadhyay, S. K., Minkina, T., Mandzhieva, S., Ranjan, A., et al. (2023a). Can nanomaterials improve the soil microbiome and crop productivity? Agriculture 13, 231. doi: 10.3390/agriculture13020231
Rajput, V. D., Singh, A., Minkina, T. M., Verma, K. K., Singh, A. K. (2023b). Nanotechnology for Sustainable Agriculture: An Innovative and Eco-Friendly Approach. 1st ed (Burlington, Canada: Apple Academic Press). doi: 10.1201/9781003333128
Rani, M., Kaushik, P., Bhayana, S., Kapoor, S. (2023). Impact of organic farming on soil health and nutritional quality of crops. J. Saudi Soc Agric. Sci. 22, 560–569. doi: 10.1016/j.jssas.2023.07.002
Rasheed, A., Li, H., Tahir, M. M., Mahmood, A., Nawaz, M., Shah, A. N., et al. (2022). The role of nanoparticles in plant biochemical, physiological, and molecular responses under drought stress: A review. Front. Plant Sci. 13. doi: 10.3389/fpls.2022.976179
Rashid, A., Schutte, B. J., Ulery, A., Deyholos, M. K., Sanogo, S., Lehnhoff, E. A., et al. (2023). Heavy metal contamination in agricultural soil: environmental pollutants affecting crop health. Agronomy 13, 1521. doi: 10.3390/agronomy13061521
Ribeiro, I. D. A., Volpiano, C. G., Vargas, L. K., Granada, C. E., Lisboa, B. B., Passaglia, L. M. (2020). Use of mineral weathering bacteria to enhance nutrient availability in crops: A Review. Front. Plant Sci. 11. doi: 10.3389/fpls.2020.590774
Rizwan, M., Ali, S., Rehman, M. Z. U., Riaz, M., Adrees, M., Hussain, A., et al. (2021). Effects of nanoparticles on trace element uptake and toxicity in plants: A review. Ecotoxicol. Environ. Saf. 221, 112437. doi: 10.1016/j.ecoenv.2021.112437
Rubin, R., Oldfield, E., Lavallee, J., Griffin, T., Mayers, B., Sanderman, J. (2023). Climate mitigation through soil amendments: quantification, evidence, and uncertainty. Carbon Manage. 14, 1. doi: 10.1080/17583004.2023.2217785
Saeed, Z., Naveed, M., Imran, M., Bashir, M. A., Sattar, A., Mustafa, A., et al. (2019). Combined use of Enterobacter sp. MN17 and zeolite reverts the adverse effects of cadmium on growth, physiology and antioxidant activity of Brassica napus. PloS One 14, e0213016. doi: 10.1371/journal.pone.0213016
Hossain, M.S. (2019). Present scenario of global salt affected soils, its management and importance of salinity research. Int. Res. J. Biol. Sci. Perspect. 1 (1), 1–3.
Seysdsharifi, R., Khoramdel, S. (2016). Effects of Nano-zinc oxide and seed inoculation by plant growth promoting Rhizobacteria (PGPR) on yield, yield components and grain filling period of soybean (Glycine max L.). Iran J. Field Crop Res. 13, 738–753. doi: 10.22067/gsc.v13i4.32491
Shah, A., Nazari, M., Antar, M., Msimbira, L. A., Naamala, J., Lyu, D., et al. (2021). PGPR in agriculture: A sustainable approach to increasing climate change resilience. Front. Sust. Food Syst. 5. doi: 10.3389/fsufs.2021.667546
Sharma, S. K., Kumari, A., Dwivedi, V., Rai, P. K., Gupta, M. (2020). Nanomaterials and Its Application in Biofuel Production. In: Eds. Srivastava, N., Srivastava, M., Mishra, P., Gupta, V. Substrate Analysis for Effective Biofuels Production. Clean Energy Production Technologies. (Singapore: Springer). doi: 10.1007/978-981-32-9607-7_10
Sharma, A., Singh, R. N., Song, X.-P., Singh, R. K., Guo, D.-J., Singh, P., et al. (2023). Genome analysis of a halophilic Virgibacillus halodenitrificans ASH15 revealed salt adaptation, plant growth promotion, and isoprenoid biosynthetic machinery. Front. Microbiol. 14. doi: 10.3389/fmicb.2023.1229955
Singh, M., Siddiqui, N., Verma, K. K. (2013). Agro-physiological traits in soybean (Glycine max L.) during salinity (Germany: Lambert Academic Publishing).
Singh, P., Singh, R. K., Li, H.-B., Guo, D.-J., Sharma, A., Lakshmanan, P., et al. (2021). Diazotrophic bacteria Pantoea dispersa and Enterobacter asburiae promote sugarcane growth by inducing nitrogen uptake and defense-related gene expression. Front. Microbiol. 11. doi: 10.3389/fmicb.2020.600417
Sonali, J. M. I., Gayathri, K. V., Rangasamy, G., Kumar, P. S., Rajagopal, R. (2023). Efficacy of biogenic selenium nanoparticles from Pseudomonas libanesis towards growth enhancement of Okra. Waste Biomass Valor 15, 1793–1806. doi: 10.1007/s12649-023-02233-1
Subotic, A., Jevremović, S., Milošević, S., Trifunović-Momčilov, M., Đurić, M., Koruga, Đ. (2022). Physiological response, oxidative stress assessment and aquaporin genes expression of cherry tomato (Solanum lycopersicum L.) exposed to hyper-harmonized fullerene water complex. Plan. Theory 11, 2810. doi: 10.3390/plants11212810
Suleimanova, A., Bulmakova, D., Sokolnikova, L., Egorova, E., Itkina, D., Kuzminova, O., et al. (2023). Phosphate solubilization and plant growth promotion by pantoea brenneri soil isolates. Microorganisms 11 (5), 1136. doi: 10.3390/microorganisms11051136
Sun, Y., Ma, L., Ma, J., Li, B., Zhu, Y., Chen, F. (2022). Combined application of plant growth-promoting bacteria and iron oxide nanoparticles ameliorates the toxic effects of arsenic in Ajwain (Trachyspermum ammi L.). Front. Plant Sci. 13. doi: 10.3389/fpls.2022.1098755
Swarnalakshmi, K., Yadav, V., Tyagi, D., Dhar, D. W., KanNepalli, A., Kumar, S. (2020). Significance of plant growth promoting rhizobacteria in grain legumes: growth promotion and crop production. Plants 9 (11), 1596. doi: 10.3390/plants9111596
Tang, L., Shi, Y., Zhang, Y., Yang, D., Guo, C. (2023). Effects of plant-growth-promoting rhizobacteria on soil bacterial community, soil physicochemical properties, and soil enzyme activities in the rhizosphere of alfalfa under field conditions. Diversity 15, 537. doi: 10.3390/d15040537
Tariq, S., Bano, A. (2023). Role of PGPR and silver nanoparticles on the physiology of Momordica charantia L. irrigated with polluted water comprising high Fe and Mn. Int. J. Phytoremed. 25, 1643–1655. doi: 10.1080/15226514.2023.2180288
Timmusk, S., Seisenbaeva, G., Behers, L. (2018). Titania (TiO2) nanoparticles enhance the performance of growth-promoting rhizobacteria. Sci. Rep. 8 (1), 617. doi: 10.1038/s41598-017-18939-x
Timofeeva, A. M., Galyamova, M. R., Sedykh, S. E. (2023). Plant growth-promoting soil bacteria: nitrogen fixation, phosphate solubilization, siderophore production, and other biological activities. Plants 12 (24), 4074. doi: 10.3390/plants12244074
Tolisano, C., Del Buono, D. (2023). Biobased: Biostimulants and biogenic nanoparticles enter the scene. Sci. Total Environ. 885, 163912. doi: 10.1016/j.scitotenv.2023.163912
Tourinho, P. S., Van Gestel, C. A., Lofts, S., Svendsen, C., Soares, A. M., Loureiro, S. (2012). Metal-based nanoparticles in soil: Fate, behavior, and effects on soil invertebrates. Environ. Toxicol. Chem. 31, 1679–1692. doi: 10.1002/etc.1880
Upadhayay, V. K., Chitara, M. K., Mishra, D., Jha, M. N., Jaiswal, A., Kumari, G., et al. (2023). Synergistic impact of nanomaterials and plant probiotics in agriculture: A tale of two-way strategy for long-term sustainability. Front. Microbiol. 14. doi: 10.3389/fmicb.2023.1133968
Urra, J., Alkorta, I., Garbisu, C. (2019). Potential benefits and risks for soil health derived from the use of organic amendments in agriculture. Agronomy 9, 542. doi: 10.3390/agronomy9090542
Verma, K. K., Li, D. M., Singh, M., Rajput, V. D., Malviya, M. K., Minkina, T., et al. (2020a). Interactive role of silicon and plant-rhizobacteria mitigating abiotic stresses: A new approach for sustainable agriculture and climate change. Plants 9, 1055. doi: 10.3390/plants9091055
Verma, K. K., Singh, P., Song, X.-P., Malviya, M. K., Singh, R. K., Chen, G.-L., et al. (2020b). Mitigating climate change for sugarcane improvement: role of silicon in alleviating abiotic stresses. Sugar Tech. 22, 741–749. doi: 10.1007/s12355-020-00831-0
Verma, K. K., Song, X. P., Joshi, A., Rajput, V. D., Singh, M., Sharma, A., et al. (2022c). Nanofertilizer possibilities for healthy soil, water and food in future: An overview. Front. Plant Sci. 13. doi: 10.3389/fpls.2022.865048
Verma, K. K., Song, X. P., Joshi, A., Tian, D. D., Rajput, V. D., Singh, M., et al. (2022d). Recent trends in nano-fertilizer for advancing sustainable agriculture under the era of climate change to ensure food security for future generations. Nanomaterials 12, 173. doi: 10.3390/nano12010173
Verma, K. K., Song, X. P., Li, D. M., Singh, M., Wu, J. M., Singh, R. K., et al. (2022b). Silicon and soil microorganisms improves rhizospheric soil health with bacterial community, plant growth, performance and yield. Plant Signaling Behav. 17, e2104004. doi: 10.1080/15592324.2022.2104004
Verma, K. K., Song, X. P., Singh, M., Tian, D. D., Rajput, V. D., Minkina, T., et al. (2023b). “Association of silicon and soil microorganisms induces stress mitigation, increasing plant productivity,” in Benefits of Silicon in the Nutrition of Plants. Ed. de Mello Prado, R. (Springer, Cham), 299–328. doi: 10.1007/978-3-031-26673-7_17
Verma, K. K., Song, X., Verma, C. L., Huang, H., Singh, M., Xu, L., et al. (2023a). Mathematical modeling of climate and fluoride effects on sugarcane photosynthesis with silicon nanoparticles. Plant Physiol. Biochem. 204, 108089. doi: 10.1016/j.plaphy.2023.108089
Verma, K. K., Song, X. P., Yadav, G., Degu, H. D., Parvaiz, A., Singh, M., et al. (2022a). Impact of agroclimatic variables on proteo-genomics in sugarcane (Saccharum spp.) plant productivity. ACS Omega 7, 22997–23008. doi: 10.1021/acsomega.2c01395
Vurukonda, S. S. K. P., Vardharajula, S., Shrivastava, M., SkZ, A. (2016). Enhancement of drought stress tolerance in crops by plant growth promoting rhizobacteria. Microbiol. Res. 184, 13–24. doi: 10.1016/j.micres.2015.12.003
Walpola, B. C., Yoon, M.-H. (2013). Phosphate solubilizing bacteria: Assessment of their effect on growth promotion and phosphorous uptake of mung bean (Vigna radiata [L.] R. Wilczek). Chilian J. Agric. Res. 73, 275–281. doi: 10.4067/S0718-58392013000300010
Wang, Y., Narayanan, M., Shi, X., Chen, X., Li, Z., Natarajan, D., et al. (2022). Plant growth-promoting bacteria in metal-contaminated soil: Current perspectives on remediation mechanisms. Front. Microbiol. 13. doi: 10.3389/fmicb.2022.966226
Watts, N., Amann, M., Ayeb-Karlsson, S., Belesova, K., Bouley, T., Boykoff, M., et al. (2018). The Lancet Countdown on health and climate change: From 25 years of inaction to a global transformation for public health. Lancet 391, 581–630. doi: 10.1016/S0140-6736(17)32464-9
Weselowski, B., Nathoo, N., Eastman, A. W., MacDonald, J., Yuan, Z.-C. (2016). Isolation, identification and characterization of Paenibacillus polymyxa CR1 with potentials for biopesticide, biofertilization, biomass degradation and biofuel production. BMC Microbiol. 16, 244. doi: 10.1186/s12866-016-0860-y
Yang, Y., Wang, J., Xiu, Z., Alvarez, P. J. (2013). Impacts of silver nanoparticles on cellular and transcriptional activity of nitrogen-cycling bacteria. Environ. Toxicol. Chem. 32, 1488–1494. doi: 10.1002/etc.2230
Zand, A. D., Tabrizi, A. M., Heir, A. V. (2020). Application of titanium dioxide nanoparticles to promote phytoremediation of Cd-polluted soil: contribution of PGPR inoculation. Bioremed. J. 2-3, 171–189. doi: 10.1080/10889868.2020.1799929
Zhao, L., Peng, B., Hernandez-Viezcas, J. A., Rico, C., Sun, Y., Peralta-Videa, J. R., et al. (2012). Stress response and tolerance of Zea mays to CeO2 nanoparticles: cross talk among H2O2, heat shock protein, and lipid peroxidation. ACS Nano 6, 9615–9622. doi: 10.1021/nn302975u
Keywords: agro-ecological responses, food security, plant-microbiome, soil amendment, NPs, PGPR
Citation: Verma KK, Joshi A, Song X-P, Singh S, Kumari A, Arora J, Singh SK, Solanki MK, Seth CS and Li Y-R (2024) Synergistic interactions of nanoparticles and plant growth promoting rhizobacteria enhancing soil-plant systems: a multigenerational perspective. Front. Plant Sci. 15:1376214. doi: 10.3389/fpls.2024.1376214
Received: 25 January 2024; Accepted: 15 April 2024;
Published: 29 April 2024.
Edited by:
Marzena Sujkowska-Rybkowska, Warsaw University of Life Sciences, PolandReviewed by:
Sumera Yasmin, National Institute for Biotechnology and Genetic Engineering, PakistanSuresh Kaushik, Independent researcher, New Delhi, India
Copyright © 2024 Verma, Joshi, Song, Singh, Kumari, Arora, Singh, Solanki, Seth and Li. This is an open-access article distributed under the terms of the Creative Commons Attribution License (CC BY). The use, distribution or reproduction in other forums is permitted, provided the original author(s) and the copyright owner(s) are credited and that the original publication in this journal is cited, in accordance with accepted academic practice. No use, distribution or reproduction is permitted which does not comply with these terms.
*Correspondence: Xiu-Peng Song, eGl1cGVuZ3NvbmdAZ3hhYXMubmV0; Yang-Rui Li, bGl5ckBneGFhcy5uZXQ=
†These authors have contributed equally to this work
‡ORCID: Yang-Rui Li, orcid.org/0000-0002-7559-9244