- 1School of Life Science and Technology, Wuhan Polytechnic University, Wuhan, China
- 2Key Laboratory of Biology and Cultivation of Chinese Herbal Medicines, Ministry of Agriculture and Rural Affairs, Enshi, China
- 3Institute of Chinese Herbal Medicines, Hubei Academy of Agricultural Sciences, Enshi, China
Cell death-inducing proteins (CDIPs) play important roles in the infection of Botrytis cinerea, a broad host-range necrotrophic phytopathogen. Here, we show that the secreted protein BcCDI1 (Cell Death Inducing 1) can cause necrosis in tobacco leaves and at the same time elicit plant defense. The transcription of Bccdi1 was induced at the infection stage. Deletion or overexpression of Bccdi1 resulted in no notable change in disease lesion on bean, tobacco, and Arabidopsis leaves, indicating that Bccdi1 has no effect on the final outcome of B. cinerea infection. Furthermore, the plant receptor-like kinases BAK1 and SOBIR1 are required to transduce the cell death-promoting signal induced by BcCDI1. These findings suggest that BcCDI1 is possibly recognized by plant receptors and then induces plant cell death.
Introduction
Botrytis cinerea is a necrotrophic fungus and typical broad host-range phytopathogen, which leads to gray mold and rot diseases in a wide range of agriculturally important crops, causing severe economic loss worldwide (Dean et al., 2012; Veloso and van Kan, 2018). To date, B. cinerea has been used as a model fungus for studying the interaction mechanism between necrotrophic pathogens and plants. However, the molecular mechanism for the pathogenicity of B. cinerea remains largely unknown.
Plants are constantly confronted with various microbial pathogens, some of which are devastating in agriculture. Plants can survive due to the development of sophisticated defense systems during the long-term coexistence with phytopathogens (Jones and Dangl, 2006). The first defense layer of plants is pathogen-associated molecular pattern (PAMP)-triggered immunity (PTI), in which the host receptors recognize the PAMPs of various pathogens and induce effective resistance responses against them. Some recent studies have demonstrated that plants can employ multiple cell surface pattern recognition receptors (PRRs) to sense and recognize the PAMPs of diverse pathogens, thereby initiating a series of intracellular signaling and then activating various immune defense responses against the pathogen (Schellenberger et al., 2019; Albert et al., 2020; Zhou and Zhang, 2020). In addition, many studies have also revealed that several of these receptors can interact with the leucine-rich repeat (LRR) receptor-like kinase (RLK) BRI1-ASSOCIATED KINASE-1 (BAK1) and SUPPRESSOR OF BIR1-1 (SOBIR1) to form signaling-competent receptor complexes required for immune signaling (van der Burgh et al., 2019).
For overcoming plant immunity and effectively infecting the host, biotrophic and hemibiotrophic pathogens generally secrete diverse effectors to the interaction area between fungal hyphae and the host or into plant cells during infection to prevent host detection or interfere with the plant defense system (Stergiopoulos and de Wit, 2009; Lo Presti et al., 2015; Kim et al., 2016). Plants have also co-evolved the effector-triggered immunity (ETI) mediated by Resistance (R) proteins, which is often associated with the hypersensitive response (HR) of local plant cells at the infection site, thereby restricting the further progression of infection by biotrophic and hemibiotrophic pathogens (Jones and Dangl, 2006; van Ooijen et al., 2007; Cui et al., 2015). However, HR and the related programmed cell death (PCD) of plants are ineffective for necrotrophic pathogens, and necrotrophs can even exploit the HR and PCD to facilitate their infection. For example, Victorin, an effector protein secreted by the host-specific necrotroph Cochliobolus victoriae, can elicit a resistance-like response in Arabidopsis thaliana and then facilitate disease progress (Lorang et al., 2007; Lorang et al., 2012). Other two host-specific necrotrophic wheat pathogens, Parastagonospora and Pyrenophora tritici-repentis, can secrete multiple necrotrophic effectors to induce severe necrosis and confer disease susceptibility in wheat genotypes harboring specific sensitivity genes (Oliver et al., 2012; Gao et al., 2015; Shi et al., 2015; Dagvadorj et al., 2022).
Eizner et al. (2017) reported that the infection process of B. cinerea on the host plant typically comprises three stages: the early stage characterized by the formation of local necrosis lesions, the intermediate stage determining the outcome of B. cinerea infection, and the late stage of fast lesion spreading. Since B. cinerea achieves its infection and colonization by killing plant cells, it secretes diverse effector proteins to manipulate the host defense and/or induce necrosis to facilitate its infection on the host plants (Heard et al., 2015; Denton-Giles et al., 2020; Bi et al., 2021; Choquer et al., 2021; Reboledo et al., 2021; Shao et al., 2021; Zhu et al., 2022). These secreted effector proteins play important roles in the pathogen–plant interaction. However, little is known about the biochemical activities and molecular mechanisms for the effect of such necrotrophic effectors.
In this study, to analyze potential B. cinerea effectors, we further identified and characterized a secreted protein BcCDI1 with cell death-inducing activity reported by Franco-Orozco et al. (2017). Our results demonstrated that BcCDI1 could activate the defense response of Nicotiana benthamiana and its necrosis-inducing signal is mediated by BAK1 and SOBIR1.
Materials and methods
Fungi, bacteria, plants, and culture conditions
The B. cinerea wild-type strain B05.10 and the derived transformants were grown and maintained on PDA medium (Acumedia, MI, USA) at 22°C under continuous fluorescent light supplemented with near-UV (black) light. The conidia of all B. cinerea strains were obtained after 7 days of culture. The Escherichia coli strains of BL21 (DE3) and DH5α (Shanghai Weidi Biotechnology, Shanghai, China) were respectively used for protein expression and plasmid construction. The Agrobacterium tumefaciens strain GV3101 (Shanghai Weidi Biotechnology, Shanghai, China) was used for Agrobacterium-mediated transient expression of target proteins in plant leaves.
Arabidopsis thaliana (Columbia-0) was grown in a greenhouse under 16-h/8-h intervals of light/dark at 22°C/20°C. Tobacco (N. benthamiana), wheat (Triticum aestivum cv. Galil), bean (Phaseolus vulgaris, genotype N9059), tomato (Solanum lycopersicum cv. Hawaii 7981), and maize (Zea mays cv. Silver Queen) plants were grown in a greenhouse under 16-h/8-h intervals of light/dark at 25°C/22°C.
Plasmid construction
The Bccdi1 deletion construct was generated as described previously (Zhu et al., 2017). The 5′ (550 bp) and 3′ (551 bp) flanking fragments of the Bccdi1 gene were amplified and respectively cloned into the upstream and downstream regions of the hph cassette using Gibson Assembly Master Mix kit (New England Biolabs, MA, USA). The overexpression plasmid was generated by cloning the full-length open reading frame of Bccdi1 into the pH2G vector under the regulation of B. cinerea histone H2B promoter (NCBI identifier CP009806.1) and the endo-β-1,4-glucanase precursor terminator (NCBI identifier CP009807.1) as described previously (Zhu et al., 2017).
For transient expression of the target protein in plants using the agroinfiltration method, the indicated sequences fused with the 3×FLAG tag were cloned into vector pCHF3 between the 2×CaMV 35S promoter and the NOS terminator and then transformed into the A. tumefaciens strain GV3101. The E. coli protein expression vector was constructed by cloning the Bccdi1 mature sequence without the signal peptide (BcCI1ΔSP) into the vector pTac-His-MBP-PreScission (Beyotime Biotechnology, Shanghai, China) and then transformed into the E. coli strain BL21 (DE3).
Manipulation of nucleic acids
The total RNA of fungi and plants was isolated using the RN03-RNApure Kit (Aidlab, Beijing, China), and the residual DNA in RNA was removed using DNase I (Thermo Scientific, MA, USA) according to protocols and stored at −80°C. The first-strand cDNA of the samples was generated using the cDNA synthesis kit (Simgen, Hangzhou, China). Reverse transcription-quantitative PCR (RT-qPCR) was performed to analyze the gene expression profile using SYBR® Green Mixes (Simgen, Hangzhou, China) and CFX96 Touch Real-Time PCR Detection System (Bio-Rad, CA, USA) according to the manufacturer’s instructions. The B. cinerea Bcgpdh gene and the N. benthamiana NbEF1α gene were respectively used as endogenous control genes to normalize the expression level of target genes as described previously (Zhu et al., 2017). For each analyzed gene, the RT-qPCR assay was repeated at least twice, and each repetition included three independent replicates. The genomic DNA of the indicated B. cinerea strains was isolated using the Fungal Genomic DNA Purification Kit (Simgen, Hangzhou, China) according to the protocol. All the primers used are listed in Supplementary Table S1.
Bioinformatics analysis
The NCBI (http://www.ncbi.nlm.nih.gov/) database was used to obtain the homologous sequences of BcCDI1 in other pathogens through a BLASTp analysis. The JGI (http://genome.jgi.doe.gov/Botci1/Botci1.home.html) database of B. cinerea was used to characterize B. cinerea genomic and transcriptomic sequences. The SMART (http://smart.embl-heidelberg.de/smart/change_mode.pl) was used to analyze the protein domain and predict signal peptide sequence. The Clustal X and MEGA-X programs were used for protein alignments and phylogenetic tree construction with an unrooted neighbor-joining method.
Transformation, pathogenicity, and cell wall stress tolerance assay of Botrytis cinerea
Genetic transformation of B. cinerea was performed as described previously (Ma et al., 2017). The Bccdi1 deletion mutant ΔBcCDI1 and the overexpression strain OEBcCDI1 were confirmed using PCR and RT-qPCR.
Pathogenicity assay on the primary leaves of 9-day-old bean or tobacco was performed as previously described (Zhu et al., 2017). The conidia of the indicated B. cinerea strains were suspended in an inoculation medium [Gamborg’s B5 medium containing 2% (w/v) glucose and 10 mM of KH2PO4/K2HPO4, pH 6.4]. Then, 8 μl of conidial suspension (1 × 105 conidia/ml) was inoculated on the leaves of the bean or tobacco, or the mycelial plugs cut from the edge of B. cinerea were inoculated on the leaves of the plants. The infected plants were incubated in a humid chamber at 22°C for 48 or 72 h, and the lesion diameter was then measured.
To analyze the possible effect of BcCDI1 on the cell wall integrity of B. cinerea, the indicated strains were inoculated on PDA plates supplemented with 0.5 mg/ml of Congo Red, 0.3 mg/ml of Calcofluor White, 1 M of sorbitol, 1 M of NaCl, and 0.02% SDS at 22°C, as described previously (Zhu et al., 2022).
Agrobacterium tumefaciens-mediated transient expression and Western blotting assay
Agrobacterium tumefaciens-mediated transient expression in N. benthamiana leaves was performed using the agroinfiltration method as previously described (Kettles et al., 2017). Extraction of the total protein of plants or fungi was performed. Approximately 0.2 g of tissue was ground to a powder using liquid nitrogen and suspended in 1 ml of cold lysis buffer (Beyotime Biotechnology, Shanghai, China). Then, the target samples were incubated on ice for 5 min and centrifuged at 12,500 rpm for 10 min at 4°C to collect the supernatant-soluble proteins. The supernatant proteins were then mixed with 5× SDS-PAGE sample buffer (Beyotime Biotechnology, Shanghai, China) and denatured by boiling for 10 min at 100°C. The denatured proteins were separated by SDS-PAGE electrophoresis and transferred onto PVDF membranes (0.45 μm). Western blotting was carried out using an anti-GFP antibody (Beyotime Biotechnology, Shanghai, China).
Expression and purification of recombinant His-MBP-BcCDI1ΔSP protein
The expression of His-MBP-BcCDI1ΔSP and His-MBP-GFP recombinant proteins was performed in the E. coli strain BL21 (DE3) as previously described (Zhu et al., 2017). Purification of recombinant proteins was performed using Glutathione Beads 4FF (Smart-Lifesciences, Changzhou, China) following the manufacturer’s instructions. The proteins were cleaned using Amicon Ultra-4 Centrifugal Filter Devices (15 ml, 10 kDa; Merck Millipore, Darmstadt, Germany) to remove the elution buffer, then dissolved in phosphate-buffered saline (PBS), and stored at –80°C.
Protein infiltration assay and induction of plant resistance by BcCDI1
To test the necrosis-inducing activity of BcCDI1 in plants, 100 μg/ml of recombinant protein produced in E. coli was infiltrated into the plant leaves using a syringe. The plants were then kept in a greenhouse at 25°C and photographed 5 days post-infiltration.
To test the plant resistance-inducing activity of BcCDI1, N. benthamiana leaves were infiltrated with 5 μg/ml of the tested protein. The infiltrated plants were kept in the greenhouse for 2 days, and the treated leaves were then inoculated with B. cinerea for an additional 2 days or used for the expression analysis of defense-related genes.
VIGS in Nicotiana benthamiana
To determine whether BcCDI1-induced plant death is associated with NbBAK1 or NbSOBIR1 in tobacco, virus-induced gene silencing (VIGS) was performed to silence the expression of NbBAK1 or NbSOBIR1 as previously described (Zhu et al., 2017). The pTRV2::GFP plasmid was used as the control. The expression level of NbBAK1 or NbSOBIR1 in gene-silenced N. benthamiana was determined by RT-qPCR analysis. Then, Bccdi1 was expressed through A. tumefaciens-mediated transient expression in the leaves of NbBAK1- or NbSOBIR1-silenced N. benthamiana. The infiltrated plants were then kept in the greenhouse at 25°C, and the necrosis development was photographed 5 days after treatment.
Results
BcCDI1 contains a hypothetical signal peptide without a known domain
Bccdi1 (BCIN06g00550) is a single-copy gene encoding 202 amino acids in B. cinerea. Bioinformatics analysis revealed that the first 19 N-terminal amino acids are the potential signal peptide and there is no transmembrane domain, indicating that BcCDI1 may be a hypothetical secreted protein. In addition, SMART MODE analysis identified no protein domain or possible function in the BcCDI1 sequence. BLAST search against the NCBI database with the BcCDI1 sequence demonstrated that all homologs of BcCDI1 are only present in Ascomycete fungi and were not found in other phyla of fungi or in bacteria or oomycetes. All BcCDI1 homologs are annotated as hypothetical proteins. Multiple sequence alignment and phylogenetic analysis demonstrated significant sequence similarity between BcCDI1 and its homologs (Supplementary Figure S1).
BcCDI1 is a secreted protein with necrosis-inducing activity
Agrobacterium tumefaciens-mediated transient expression (agroinfiltration) was used to test the necrosis-inducing activity of candidate effector proteins in N. benthamiana leaves. The transient expression of full-length BcCDI1 could cause necrosis in N. benthamiana leaves (Figure 1), whereas that of the control plasmid or BcCDI1ΔSP without the predicted secretion signal peptide did not trigger leaf death (Figure 1). Since the function of the signal peptide is to guide the protein into the plant apoplastic space after transient expression in plant cells and the first 19 N-terminal amino acids are predicted to be the signal peptide, it could be inferred that BcCDI1 may be a hypothetical secreted protein that functions in the leaf apoplastic space to induce plant cell death under the guidance of the signal peptide.
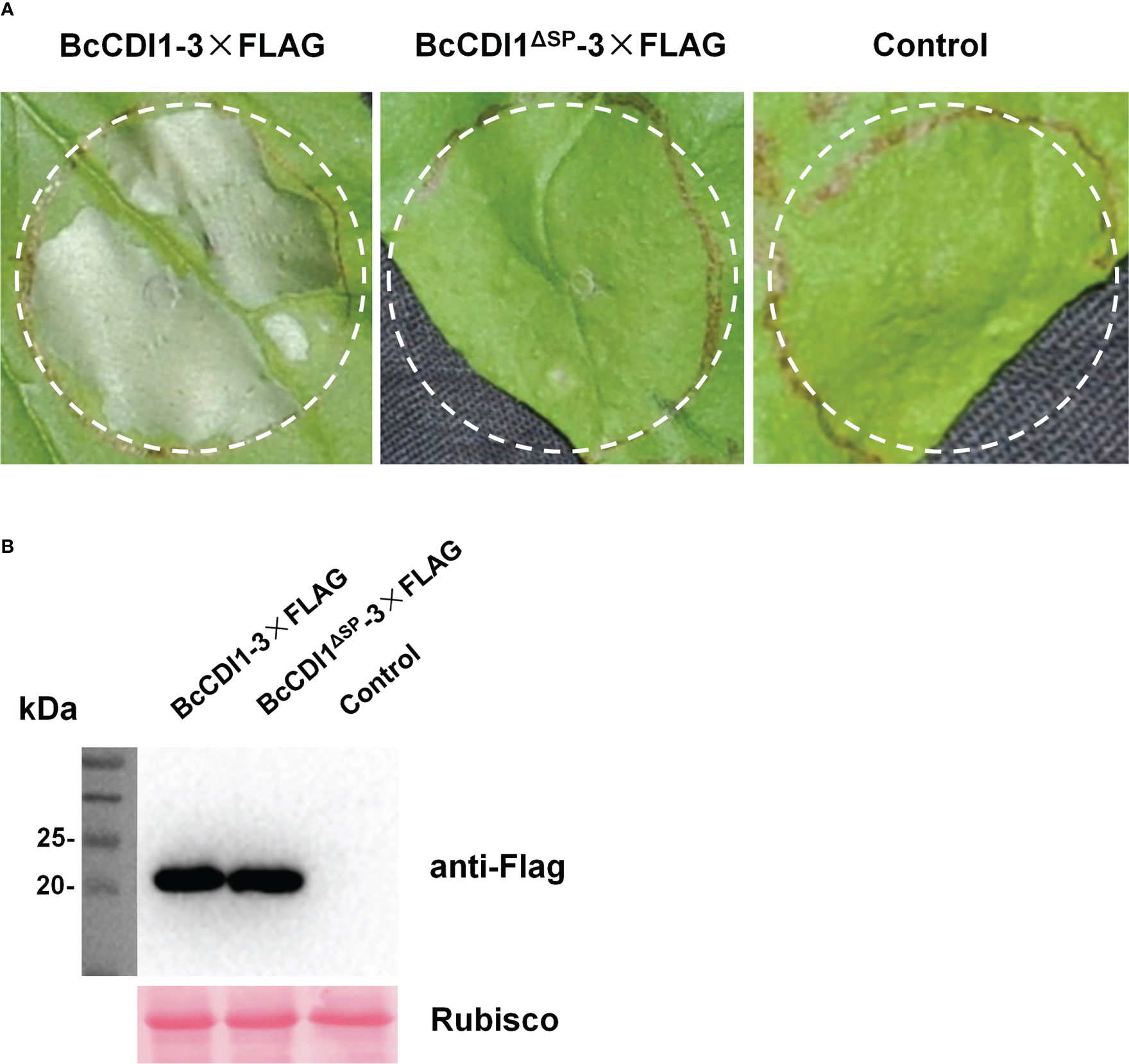
Figure 1 Full-length BcCDI1 can induce cell death, and the signal peptide is required for its function. (A) Images of Nicotiana benthamiana leaves at 5 days after agroinfiltration with Agrobacterium tumefaciens strains carrying the indicated constructs. Agrobacterium tumefaciens carrying the empty vector was used as the control. BcCDI1-3×Flag, BcCDI1 fused with 3×Flag at the C-terminus; BcCDI1ΔSP-3×Flag, BcCDI1 without the signal peptide fused with 3×Flag at the C-terminus. (B) Immunoblot analysis of proteins from N. benthamiana leaves with transient expression of the indicated constructs. Top panel, immunoblot using anti-Flag antibody; bottom panel, staining of the Rubisco large subunit with Ponceau S.
Two methods were further used to confirm whether BcCDI1 is indeed a secreted protein. First, the signal peptide of BcCDI1 was fused with GFP to generate SPBcCDI1-GFP. Similarly, we fused the signal peptide of BcXYG1, a strong cell death-inducing secreted protein of B. cinerea that functions in the apoplastic space (Zhu et al., 2017), with GFP to generate SPBcXYG1-GFP as the positive control, and the GFP overexpression construct without the signal peptide was used as the negative control. All the constructs were transformed into the wild-type B. cinerea strain to generate overexpression strains of the fused proteins. Western blot analysis showed that all the indicated proteins were successfully expressed in the hyphae of each strain (Figure 2A). Then, all examined strains were respectively cultured in potato dextrose broth (PDB) liquid medium for 3 days, and an immunological analysis was performed to check the presence of GFP in the culture filtrate. The results confirmed the accumulation of GFP in the culture medium of SPBcCDI1-GFP and SPBcXYG1-GFP overexpression strains, but not in that of GFP overexpression or wild-type strains (Figure 2B). For the second method, BcCDI1 and BcCDI1ΔSP were fused with GFP to generate transient expression plasmids of BcCDI1-GFP and BcCDI1ΔSP-GFP. Within 2 days after agroinfiltration, Western blot analysis successfully detected BcCDI1-GFP but not BcCDI1ΔSP-GFP in apoplastic fluid from infiltrated tobacco leaves (Figure 2C), indicating that BcCDI1-GFP was indeed secreted into the apoplastic space of plant cells. In summary, all these results confirmed that BcCDI1 is a secreted protein.
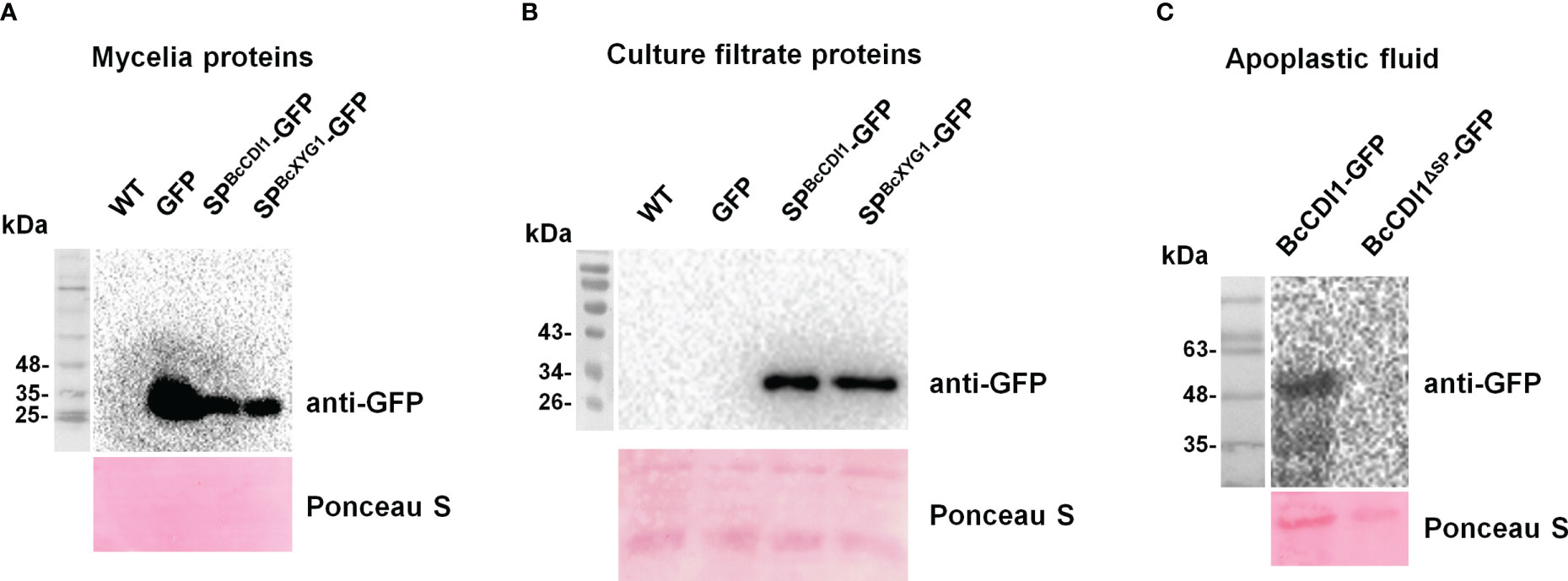
Figure 2 BcCDI1 signal peptide possesses secretion function. (A) Immunoblot analysis of the total mycelial proteins from the indicated Botrytis cinerea strains. Top panel, immunoblot using anti-GFP antibody; bottom panel, Ponceau S staining of the total mycelial proteins. (B) Immunoblot analysis of culture filtrate proteins from the indicated B cinerea strains. Top panel, immunoblot using anti-GFP antibody; bottom panel, Ponceau S staining of the secreted proteins. SPBcCDI1-GFP, GFP fused with the signal peptide of BcCDI1; SPBcXYG1-GFP, GFP fused with the signal peptide of BcXYG1; GFP, GFP overexpression strain; WT, wild-type strain. (C) Western blotting analysis of apoplastic fluid from Nicotiana benthamiana leaves agroinfiltrated with BcCDI1-GFP or BcCDI1ΔSP-GFP for 3 days. Top panel, immunoblot using anti-GFP antibody; bottom panel, Ponceau S staining of the apoplastic fluid.
BcCDI1ΔSP induces cell death in tobacco, tomato, and potato
To determine whether BcCDI1 can induce necrosis in plants besides N. benthamiana and to avoid the A. tumefaciens incompatibility effects on plants, we generated the His-MBP-BcCDI1ΔSP protein in E. coli (Supplementary Figure S2) and infiltrated tobacco leaves with the purified protein at different concentrations using a syringe. As a result, 10 μg/ml of His-MBP-BcCDI1ΔSP recombinant protein was sufficient to trigger leaf necrosis in N. benthamiana (Figure 3A). The purified His-MBP-BcCDI1ΔSP recombinant protein could also trigger cell death in tomato and potato leaves, but not in bean and A. thaliana, or in the monocots wheat and maize (Figure 3B).
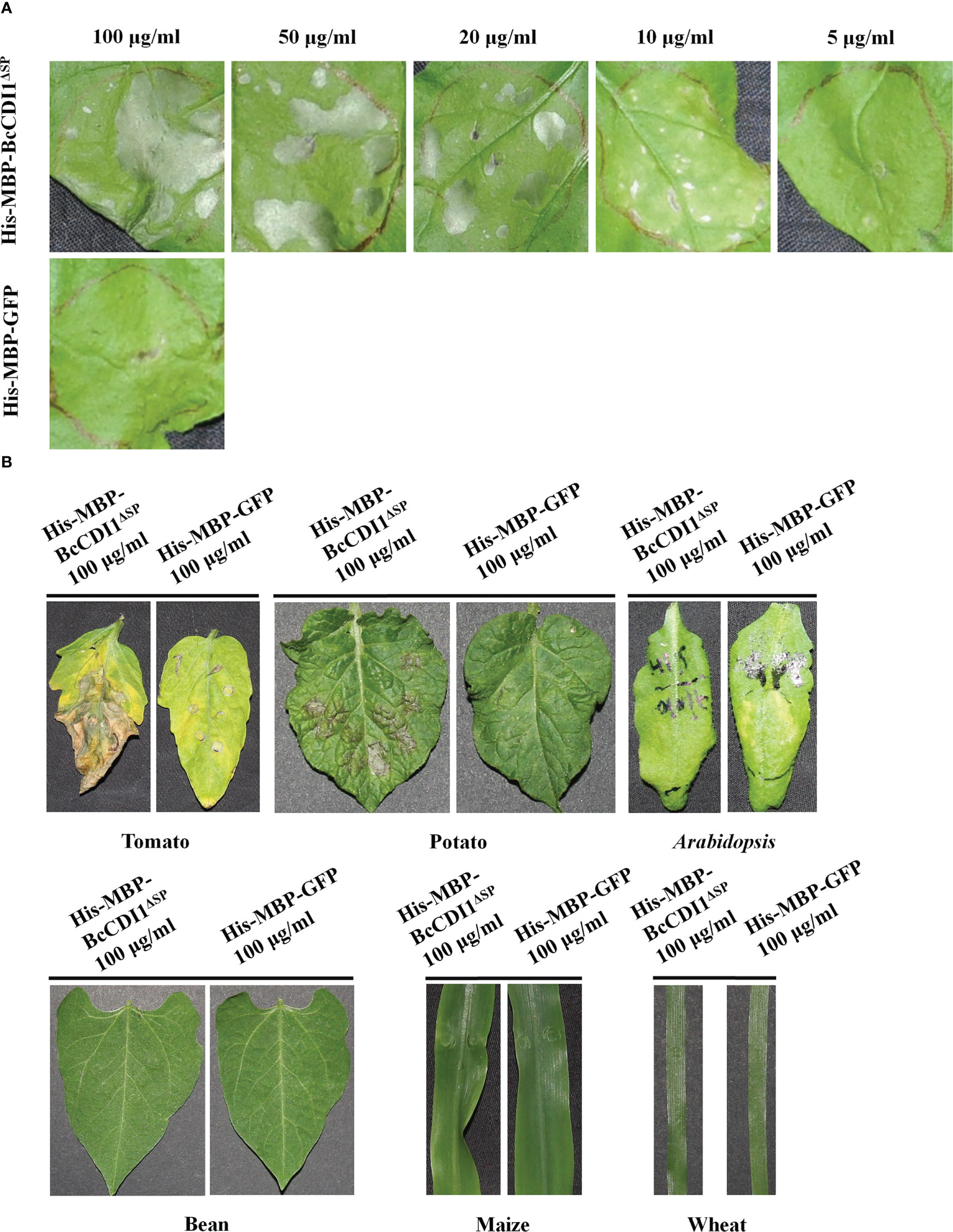
Figure 3 His-MBP-BcCDI1ΔSP protein induces necrosis in tobacco, tomato, and potato. Proteins were produced in Escherichia coli, purified, and suspended in PBS. (A) Response of Nicotiana benthamiana leaves infiltrated with different concentrations of His-MBP-BcCDI1ΔSP at 5 days after treatment. (B) Response of tomato, potato, Arabidopsis, bean, maize, and wheat leaves infiltrated with 100 μg/ml of protein solution at 5 days after treatment. The His-MBP-GFP protein was used as the control.
Bccdi1 is induced during infection but is not essential for stress tolerance and pathogenicity
RT-qPCR was carried out to determine the expression pattern of Bccdi1. As a result, the transcript level of Bccdi1 increased after inoculation on the bean leaves and reached the peak at 36 h post-inoculation (hpi), which was approximately 35-fold that at 0 hpi (Figure 4). When B. cinerea was inoculated on solid Gamborg’s B5 medium, the transcript level of Bccdi1 remained stable, and the maximum level was only approximately 12-fold that at 0 hpi (Figure 4).
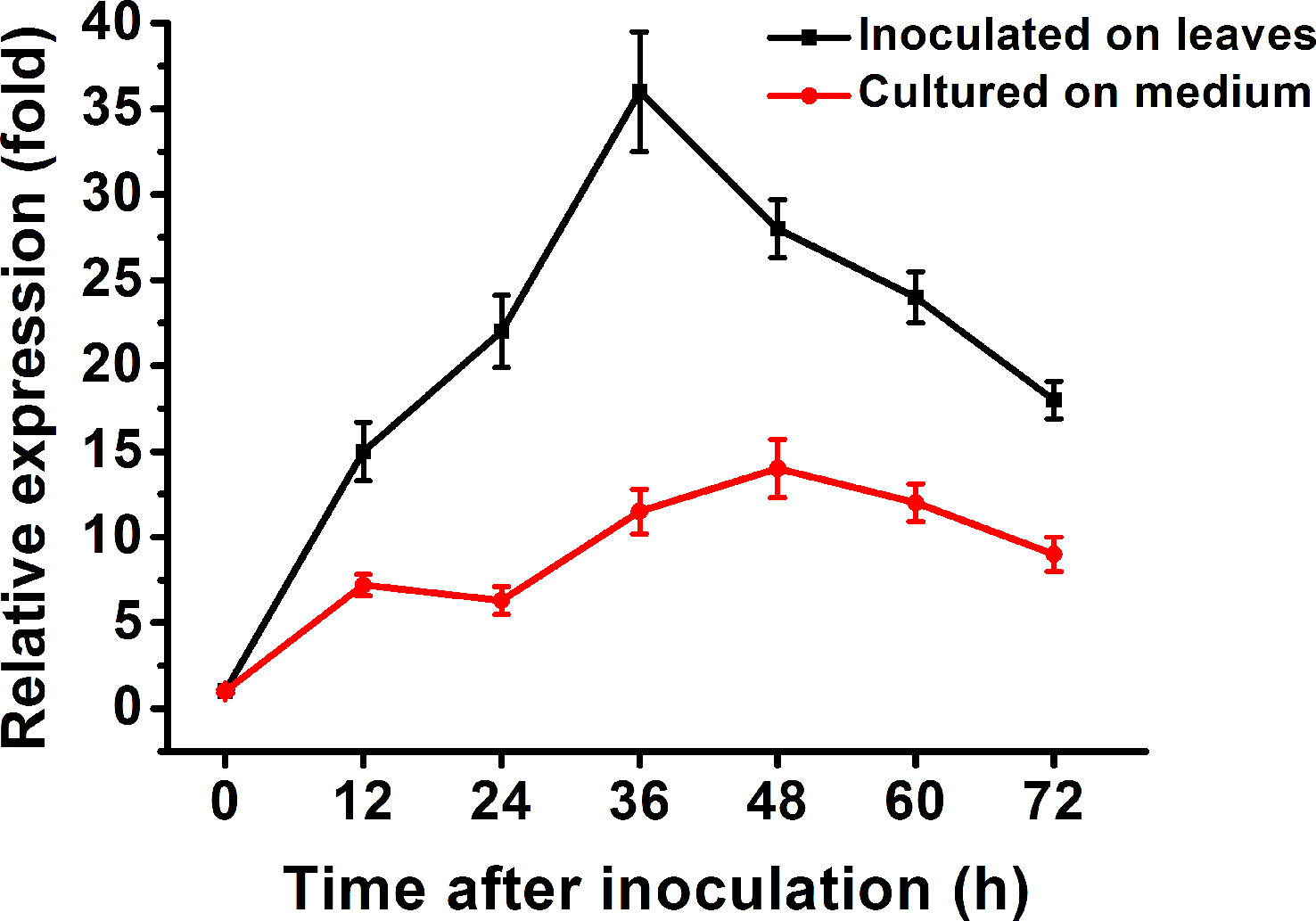
Figure 4 Expression of the Bccdi1 gene is upregulated during infection. Bean leaves (black line) or Gamborg’s B5 medium (red line) was inoculated with Botrytis cinerea conidia, and the expression level of the Bccdi1 gene was evaluated by RT-qPCR. The expression level of Bccdi1 inoculated on the plant or in Gamborg’s B5 medium at 0 h was set as 1, and the relative transcript level was calculated using the comparative Ct method. The transcript levels of the B cinerea bcgpdh gene were used to normalize different samples. Data represent means and standard deviations of three independent replications.
To further clarify the potential role of BcCDI1 in B. cinerea infection, transformants with the deletion or overexpression of Bccdi1 were generated and verified by PCR and RT-qPCR analyses (Supplementary Figure S3).
All Bccdi1 deletion and overexpression transformants exhibited normal conidial production, colony morphology, and growth rate on potato dextrose agar (PDA) as the wild-type strain (Supplementary Figure S4). In addition, no statistically significant difference in stress tolerance (0.5 mg/ml of Congo Red, 0.3 mg/ml of Calcofluor White, 1 M of NaCl, 0.02% SDS, and 1 M of sorbitol) was observed between the transformants and wild-type strain (Supplementary Figure S5).
The pathogenicity assay revealed that compared with the wild-type strain, the Bccdi1 deletion or overexpression strains had no significant difference in lesion size on bean, tobacco, and A. thaliana leaves (Figure 5), indicating that Bccdi1 has no effect on the outcome of B. cinerea infection.
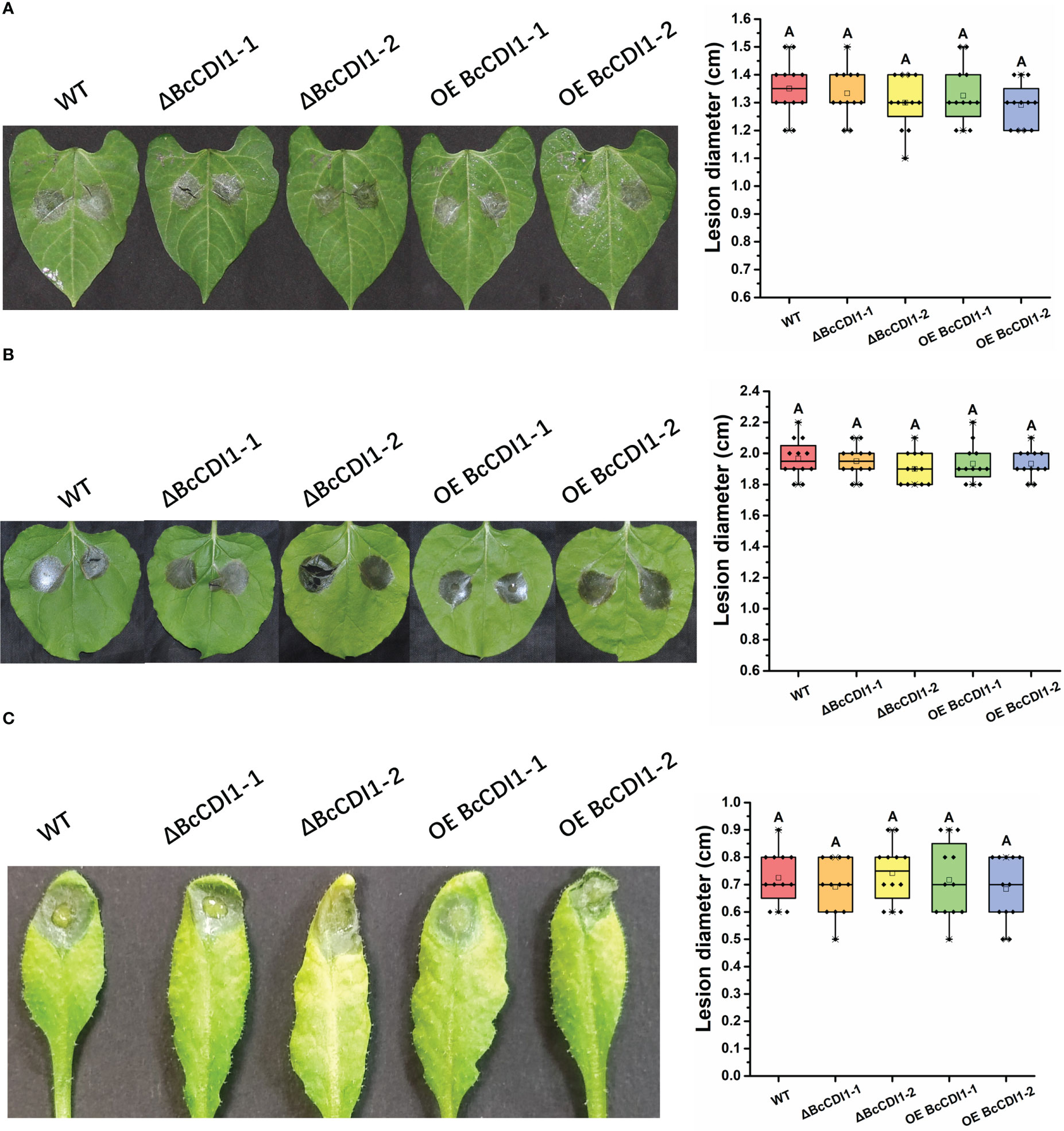
Figure 5 Pathogenicity analysis of Botrytis cinerea strain. (A) Bean leaves were inoculated with 7.5 μl of conidial suspension (1 × 105 conidia/ml). The plants were incubated in a humid chamber at 22°C for 72 h and photographed, and the lesion size was determined. (B) Tobacco leaves were inoculated with 7.5 μl of conidial suspension (1 × 105 conidia/ml). (C) Arabidopsis thaliana leaves were inoculated with 7.5 μl of conidia suspension (1 × 105 conidia/ml). The plants were incubated in a humid chamber at 22°C for 72 h and photographed, and the lesion size was determined. Data were obtained from three independent experiments, with four replicates in each experiment. In box plots, whiskers indicate the minimum and maximum values, the line indicates the median, and the box boundaries indicate the upper (25th percentile) and lower (75th percentile) quartiles. All data are plotted as black dots. Similar letters in the graph indicate no statistical differences at P ≤0.01 using ANOVA (one-way) followed by Tukey’s post-hoc test.
BcCDI1 induces the resistance of N. benthamiana against B. cinerea
Recently, several studies have revealed that some necrosis-inducing proteins can be recognized by the plant immune system and activates defense response. To determine whether BcCDI1 can trigger plant resistance, 5 μg/ml of purified His-MBP-BcCDI1ΔSP protein or His-MBP-GFP was injected into N. benthamiana leaves using a syringe. After 48 h, the infiltrated leaves were inoculated with B. cinerea and incubated in a chamber for an additional 48 h. The results showed that tobacco leaves pretreated with His-MBP-BcCDI1ΔSP had an obviously smaller lesion size than those pretreated with His-MBP-GFP protein (Figure 6A), suggesting that the BcCDI1 protein can induce plant resistance to B. cinerea infection.
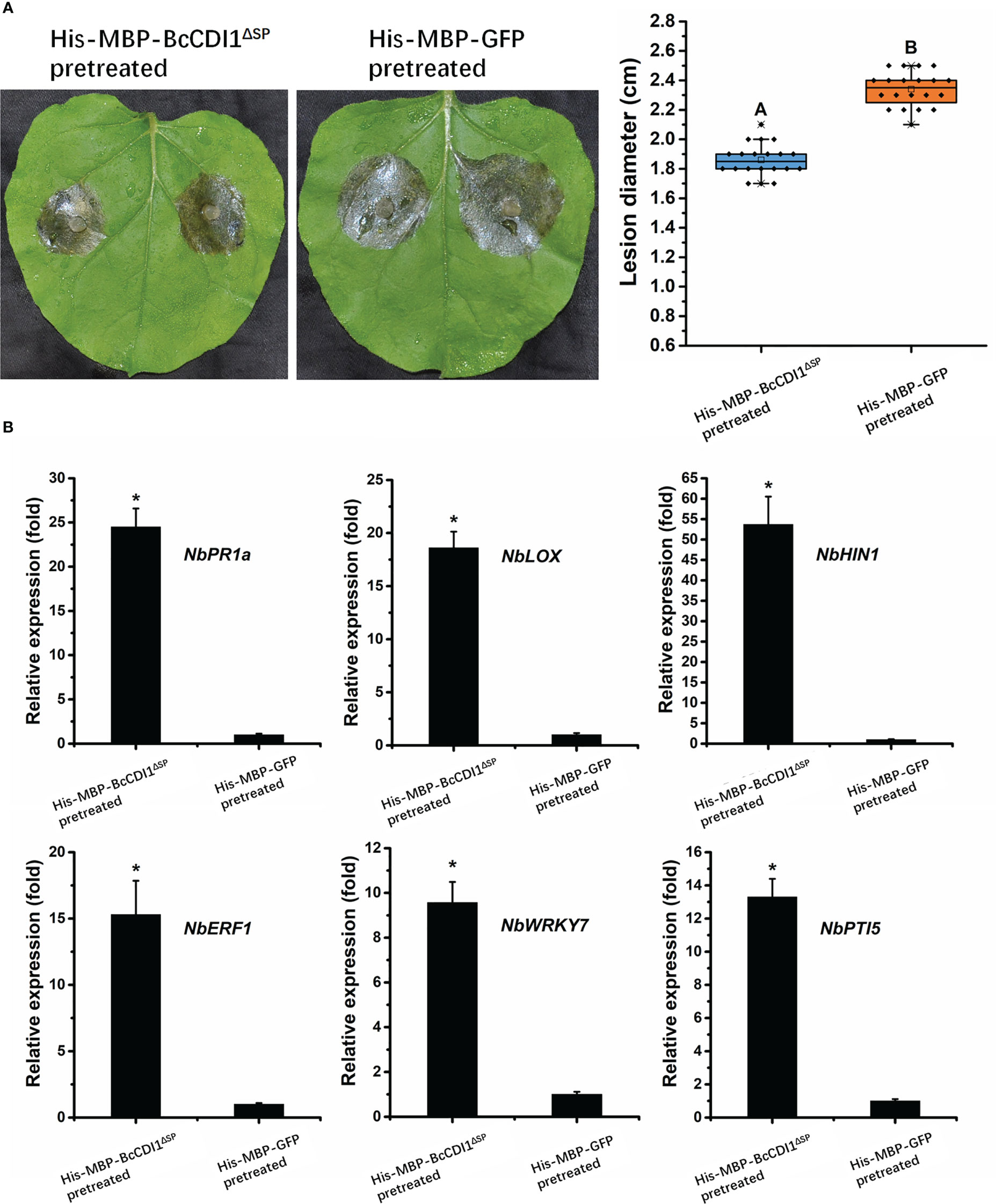
Figure 6 BcCDI1 induces resistance in tobacco. (A) Nicotiana benthamiana leaves were infiltrated with 5 μg/ml of purified BcCDI1 or GFP protein. After 2 days, the infiltrated leaves were inoculated with Botrytis cinerea in a humid chamber. The lesions were photographed and measured at 48 hpi. Data were obtained from three independent experiments with a total of 20 samples. In box plots, whiskers indicate the minimum and maximum values; the line indicates the median; the box boundaries indicate the upper (25th percentile) and lower (75th percentile) quartiles. All data are plotted as black dots. Different letters in the graph indicate statistical differences at P ≤0.01 using ANOVA (one-way) followed by Tukey’s post-hoc test. (B) The relative expression levels of defense-related genes from tobacco leaves treated with BcCDI1 or GFP for 48 h were determined by RT-qPCR analysis. The expression level of the indicated genes in GFP-treated leaves was set as 1. The expression level of the tobacco NbEF1α gene was used to normalize different samples. Data represent means and standard deviations of three independent replicates. Asterisks in the graph indicate statistical differences at P ≤0.01 using ANOVA (one-way) followed by Tukey’s post-hoc test.
To test whether the promoting effect of His-MBP-BcCDI1ΔSP on the resistance of tobacco is associated with changes in the expression of defense-related genes, RT-qPCR was performed to analyze the changes in the transcripts of the salicylic acid (SA) signal pathway gene NbPR1a, the jasmonic acid (JA) signal pathway gene NbLOX, the HR-related gene HIN1, the ethylene signal pathway gene NbERF1, and the PTI-related genes NbWRKY7 and NbPTI5 as previously described (Zhu et al., 2022). The results demonstrated that the transcripts of these defense-related genes in N. benthamiana leaves dramatically increased after infiltration of the His-MBP-BcCDI1ΔSP protein for 24 h compared with those in His-MBP-GFP-infiltrated leaves (Figure 6B). A similar result was reported in a previous study, in which the homolog of BcCDI1 in Rhynchosporium commune could also induce the transcriptional upregulation of defense-related genes in N. benthamiana leaves (Franco-Orozco et al., 2017).
We also determined whether BcCDI1 can trigger systemic resistance in non-treated leaves of the same tobacco plants. The leaves were infiltrated with 100 μg/ml of purified His-MBP-BcCDI1ΔSP or His-MBP-GFP protein. After 2 days, the untreated leaves of the same plant were inoculated with B. cinerea, and the plant was incubated in a humid chamber for an additional 48 h. As a result, the His-MBP-BcCDI1ΔSP pretreated plants showed no obvious difference in disease lesion from the His-MBP-GFP pretreated plants (Supplementary Figure S6), indicating that His-MBP-BcCDI1ΔSP cannot induce plant systemic resistance.
BAK1 and SOBIR1 are required for the necrosis-inducing activity of BcCDI1 in Nicotiana benthamiana
Since BcCDI1 is a secreted effector localized in the apoplastic space, it may interact with plant membrane RLKs or receptor-like proteins (RLPs) to transduce the immunity signals via the RLP–SOBIR1–BAK1 complex into the intracellular space, which is similar to the function of other apoplast-localized effector proteins (Liebrand et al., 2013; Zhang et al., 2014; Albert et al., 2015; Ma et al., 2015; Postma et al., 2016; Gui et al., 2017; Zhu et al., 2017). Therefore, we generated NbBAK1- and NbSOBIR1-silenced N. benthamiana using VIGS, which were then agroinfiltrated with Agrobacterium harboring BcCDI1 expression construct. The results showed that BcCDI1 failed to cause necrosis in BAK1- or SOBIR1-silenced tobacco leaves, whereas the control plant leaves infiltrated with pTRV2-GFP showed severe cell death after agroinfiltration of BcCDI1 or infiltration of purified His-MBP-BcCDI1ΔSP protein (Figure 7), indicating that both BAK1 and SOBIR1 can mediate the necrosis-inducing activity of BcCDI1, possibly through an unknown RLK or RLP.
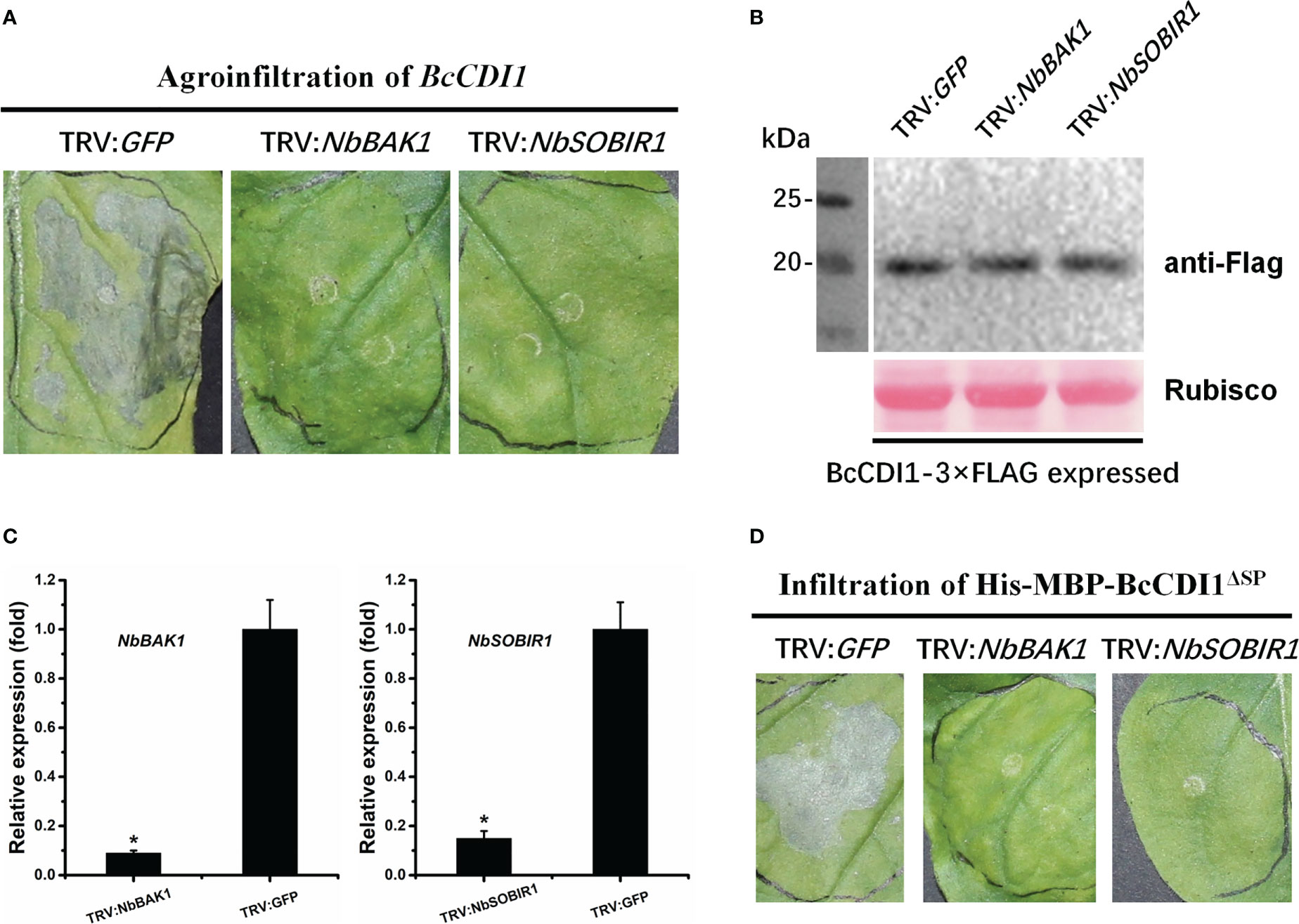
Figure 7 The necrosis-inducing activity of BcCDI1 is mediated by NbBAK1 and NbSOBIR1. (A) Three weeks after the initiation of VIGS, BcCDI1-3×Flag was transiently expressed in the gene-silenced leaves using agroinfiltration. The leaves were photographed 5 days after treatment. (B) Immunoblot analysis of proteins from the indicated Nicotiana benthamiana leaves with transient expression of BcCDI1-3×Flag. Top panel: BcCDI1-3×Flag was detected using the anti-Flag antibody; bottom panel, staining of the Rubisco large subunit with Ponceau S. (C) NbBAK1 and NbSOBIR1 expression levels in gene-silenced tobacco leaves were determined by RT-qPCR analysis. The expression level in the control plants (TRV: GFP) was set as 1. NbEF1α was used as an endogenous control. Data represent means and standard deviations from three biological replicates. Asterisks indicate significant differences (P ≤ 0.01) followed by Tukey’s post-hoc test. (D) Response of gene-silenced leaves infiltrated with 100 μg/ml of protein solution at 5 days after treatment.
Discussion
Plant pathogens can secrete effectors to promote host colonization through diverse molecular mechanisms. In B. cinerea, many predicted secreted proteins have been mined through comparative transcriptomics, secreted proteomics, or genomics approaches (Zhu et al., 2017; Mousavi-Derazmahalleh et al., 2019; Valero-Jiménez et al., 2019; Denton-Giles et al., 2020; Zhang et al., 2020; Jeblick et al., 2023). However, due to the high functional redundancy of virulence factors, only a limited number of secreted proteins have been confirmed to have a true contribution to the pathogenesis of B. cinerea (Brito et al., 2006; Frías et al., 2011; Frías et al., 2016; Zhu et al., 2017; Bi et al., 2022; Leisen et al., 2022). In the present study, putative secreted proteins predicted from B. cinerea genomics data were screened by transient expression using the agroinfiltration assay. A secreted protein, BcCDI1, was identified as a candidate effector protein involved in the invasion of B. cinerea, which could simultaneously cause necrosis and induce host resistance in tobacco.
Expression analysis revealed that the expression of Bccdi1 was upregulated at the early infection stage (0-36 hpi) of B. cinerea, reaching the peak at 36 hpi and declining thereafter. It has been demonstrated that the successful establishment of pathogen infection largely depends on the killing of a sufficient number of host cells to create a region of dead plant tissue, in which fungal biomass will be accumulated before the transition to the intermediate and then late infection phases (Shlezinger et al., 2011; Eizner et al., 2017). The specific activation of Bccdi1 in planta and induction of transcription at the early infection stage (Figure 4) indicate that BcCDI1 belongs to the array of CDIPs in B. cinerea. However, despite the evidently important role of BcCDI1 in B. cinerea pathogenicity, the deletion or overexpression transformants showed no significant difference in lesion size from the wild-type strain (Figure 5), which is not surprising considering the high functional redundancy of virulence factors in the fungal secretome (Zhu et al., 2017; Leisen et al., 2022). Indeed, similar conclusions have been reported in the analysis of other B. cinerea CIDPs, such as BcNEP1/2 (Arenas et al., 2010), BcIEB1 (Frías et al., 2016), BcXYG1 (Zhu et al., 2017), and BcCrh1 (Bi et al., 2021). Previous studies with the assistance of the PathTrack© system (Eizner et al., 2017) discovered earlier and more intense local necrosis caused by BcXYG1 overexpression strains. However, the lesion size showed no notable difference from that of the wild-type strain at the later infection stage, which can explain the similar lesion size at 72 hpi (Zhu et al., 2017). Hence, a more detailed analysis of pathogenicity, such as the use of PathTrack©, will better reveal the possible role of BcCDI1 at the early infection stage.
Recently, there has been increasing evidence suggesting that the defense stimulation of these CDIPs is not related to the necrosis-inducing activity (Oome et al., 2014; Zhu et al., 2017; Bi et al., 2021), suggesting that different plant receptors may be involved in host immune response and necrosis-inducing pathways. Necrotrophic fungi cause significant crop loss worldwide. Hence, it may be feasible to improve engineering crops with broad and durable disease resistance without damaging the host cells based on mutant CDIPs from B. cinerea that have no necrosis-inducing activity. Further research is needed to determine the relationship between necrosis-inducing activity and host defense-triggering activity.
Effector repertoire can be defined into two categories: the effectors either remain at the plant–pathogen interface (termed apoplastic effectors) or are taken up by host cells (translocated or cytoplasmic effectors) (Asai and Shirasu, 2015). To date, over 15 CDIPs have been characterized in B. cinerea. Most of them remain in the apoplastic space after secretion by the fungus, except for the transglycosylase BcCrh1, which causes plant cell death depending on cytoplasmic localization (Bi et al., 2022). The BcCDI1 protein reported here belongs to the apoplast-localized CDIPs (Figure 2), whose cell death-inducing activity is mediated by plant extracellular membrane components. Plants employ RLKs and RLPs, which are categorized according to the presence or absence of a cytoplasmic kinase domain, as PRRs to monitor their apoplastic environment and sense non-self and damaged-self patterns as signs of potential danger. BAK1 and SOBIR1 have been described as the most widespread co-receptors during the last decade of inward signaling through RLKs and RLPs (Boutrot and Zipfel, 2017). Our results demonstrated that BcCDI1 is an apoplast-localized CDIP, and its necrosis-inducing activity is dependent on NbBAK1/NbSOBIR1 and possibly mediated by an unknown RLK or RLP. Similarly, it has been reported that the necrosis-inducing activity of the BcCDI1 homolog in R. commune is also dependent on NbBAK1 and NbSOBIR1 (Franco-Orozco et al., 2017). Further studies can be conducted to improve our understanding of the molecular details involved in the BAK1- and SOBIR1-dependent recognition of BcCDI1.
To develop new strategies for controlling plant diseases caused by pathogenic fungi, it is important to fully understand the pathogenic mechanisms of fungi, the evolution of virulence traits, the molecular basis for host adaptation, and particularly how to engineer and improve the crop resistance in a more sustainable way (Doehlemann et al., 2017). The panoramic view of the invasion model and virulence factors of B. cinerea has been intensively studied. Although the specific molecular mechanisms underlying B. cinerea invasion remain elusive, it is becoming increasingly evident that the infection process of B. cinerea is far more sophisticated and complex than previously estimated.
In conclusion, exploration of the underlying pathogenic mechanisms of BcCDI1, an effector from B. cinerea, paves the way for the development of novel strategies to control this devastating disease.
Conclusions
This study characterized the secreted protein BcCDI1 from B. cinerea. Our results demonstrate that the BcCDI1 gene does not affect the outcome of B. cinerea infection or other phenotypes including conidial production, colony morphology, growth rate, and stress tolerance. Further analysis demonstrated that BcCDI1 can induce plant cell death by interacting with an unknown RLP in the apoplastic space of plant cells to transduce the immunity signals via the RLP–SOBIR1–BAK1 complex into the intracellular space and trigger plant immune response. Our findings suggest that BcCDI1 has the potential to be applied in breeding crops with resistance to gray mold and other destructive pathogenic microbes.
Data availability statement
The datasets presented in this study can be found in online repositories. The names of the repository/repositories and accession number(s) can be found in the article/Supplementary Material.
Author contributions
Conceived and designed the experiments: WZ, HD, and KB. Performed the experiments: WZ, HD, RX, and JY. Analyzed the experiment data: WZ, HD, CX, D-zY, JW, RX, and JY. Contributed reagents/materials/analysis tools: WZ, RX, JY, and KB. Wrote the paper: WZ, RX, JY, and KB. All authors contributed to the article and approved the submitted version.
Funding
This research was supported by the National Natural Science Foundation of China (Grant Nos. 31972215, 31861143043, and 81903782) and the Open Fund (Grant No. 2022ZTSJJ6) from the Key Laboratory of Integrated Pests Management on Crops in Central China/Hubei Key Laboratory of Crop Diseases, Insect Pests and Weeds Control.
Acknowledgments
We thank Dr. Wei Wei and Professor Weidong Chen at Washington State University for improving the language of the manuscript. We would also like to acknowledge the reviewers for their work on this paper.
Conflict of interest
The authors declare that the research was conducted in the absence of any commercial or financial relationships that could be construed as a potential conflict of interest.
Publisher’s note
All claims expressed in this article are solely those of the authors and do not necessarily represent those of their affiliated organizations, or those of the publisher, the editors and the reviewers. Any product that may be evaluated in this article, or claim that may be made by its manufacturer, is not guaranteed or endorsed by the publisher.
Supplementary material
The Supplementary Material for this article can be found online at: https://www.frontiersin.org/articles/10.3389/fpls.2023.1136463/full#supplementary-material
Supplementary Figure 1 | Sequence similarities between BcCDI1 and its homologues. (A) Multiple sequence alignment of BcCDI1 and its homologues. Full-length protein sequences were aligned using Clustal W and the alignment was edited using Jalview. Intensity of blue shading reflects the level of amino acid identity at each position. Bc: B. cinerea BcCDI1 (BCIN06g00550); Zt: Zymoseptoria tritici (XP_003847964.1, E-value: 5.58e-016, 48.8% identity); Uv: Ustilaginoidea virens (KDB13604.1, E-value: 2.27e-034, 55.2% identity); Rc: Rhynchosporium commune (KX499545, E-value: 2.93e-039, 45.2% identity); Ss: Sclerotinia sclerotiorum (XP_001589511.1, E-value: 2.41e-049, 74.7% identity); Pn: Parastagonospora nodorum (XP_001792149.1, E-value: 2.78e-021, 43.5% identity); Bg: Blumeria graminis f. sp. hordei (CCU82201.1, E-value: 2.25e-021, 39% identity); Cg: Colletotrichum graminicola (CAQ16238.1, E-value: 2.49e-019, 40.4% identity). (B) Phylogenetic analysis of BcCDI1 and its homologues from other fungi. The full-length protein sequences were analyzed using MEGA X with Unrooted neighbor-joining bootstrap (1000 replicates). The black circle marks the location of BcCDI1. A scale bar at the lower left corresponds to a genetic distance of 0.2.
Supplementary Figure 2 | Expression of recombinant proteins. SDS-PAGE analysis of purified His-MBP-BcCDI1ΔSP and His-MBP-GFP proteins from E. coli stained with Coomassie Blue.
Supplementary Figure 3 | Deletion and verification of the Bccdi1 gene in B. cinerea. (A) Strategy used to generate the Bccdi1 gene deletion mutants. The deletion construct used to transform the wild-type strain contained the hygromycin resistance (hph) cassette flanked by upstream and downstream sequence of the Bccdi1 gene. The positions of the PCR primers used to verify the deletion transformants are indicated (Bccdi1 Del-up F and Bccdi1 Del-down R). The scale bar indicates 500 bp. (B) PCR amplification to verify the Bccdi1 deletion mutants using the 5’flank For and 3’flank Rev primers. As templates, genomic DNA from either the wild-type strain or the Bccdi1 deletion mutants ΔBcCDI1-1 and ΔBcCDI1-2 was used as indicated. (C) RT-qPCR was carried out to analyze the expression levels of the Bccdi1 gene in the wild-type strain, the Bccdi1 deletion mutants ΔBcCDI1-1 and ΔBcCDI1-2, and the Bccdi1 overexpression strains OE BcCDI1-1 and OE BcCDI1-2. The relative transcript levels were calculated using the comparative Ct method. The Bccdi1 gene expression level in the wild-type strain was set as 1. The transcript level of the B. cinerea bcgpdh gene was used to normalize different samples. Data represent means and standard deviations of three independent replicates. ND = not detected.
Supplementary Figure 4 | Phenotypes of wild-type, and Bccdi1 deletion and overexpression strains of B. cinerea. (A) Colony morphology, sporulation, and sclerotia formation. Top: colonies on PDA at 22°C for 15 d in complete darkness. Bottom: B. cinerea strains on PDA plates at 22°C for 7 d with continuous fluorescent light. (B) Hyphal growth rate. B. cinerea strains were grown on PDA plates at 22°C with continuous fluorescent light. Radial growth was measured every day for 4 d and the growth rate was calculated. Data represent means and standard deviations of three independent replicates. Same letters in the graph indicate no statistical difference at P ≤ 0.01 using one-way ANOVA followed by Tukey’s post hoc test. (C) Conidial production of indicated strains cultured on PDA plates at 22°C for 7 d. Conidiation of each strain was determined by collecting and counting conidia with a hematocytometer. Data represent means and standard deviations of three independent replicates. Same letters in the graph indicate no statistical difference at P ≤ 0.01 using one-way ANOVA followed by Tukey’s post hoc test. Same letters in the graph indicate no statistical difference at P ≤ 0.01 using one-way ANOVA followed by Tukey’s post hoc test.
Supplementary Figure 5 | Bccdi1 deletion does not affect stress tolerance of B. cinerea. Inhibition rate of the radial growth of the wild-type strain, Bccdi1 deletion mutants and Bccdi1 overexpression strains was analyzed on PDA plates supplemented with 0.5 mg/ml Congo Red, 0.3 mg/ml Calcofluor White, 1 M NaCl, 0.02% SDS and 1 M sorbitol at 22°C, respectively. Data represent means and standard deviations from three independent experiments, each with three replications. Same letters in the graph indicate no statistical differences at P ≤ 0.01 using ANOVA (one-way) followed by Tukey’s post hoc test.
Supplementary Figure 6 | BcCDI1 does not induce systemic resistance in tobacco. N. benthamiana leaves were infiltrated with 100 μg/ml purified His-MBP-BcCDI1ΔSP or His-MBP-GFP protein. After 2 d, the non-treated leaves of the same plant were inoculated with B. cinerea in a humid chamber. The lesions were photographed and measured at 48 hpi. All data were obtained from three independent experiments with a total of 12 samples. In box plots, whiskers indicate the minimum and maximum values; the line indicates the median; the box boundaries indicate the upper (25th percentile) and lower (75th percentile) quartiles. All data are plotted as black dots. The same letters in the graph indicate no statistical difference at P ≤ 0.01 using ANOVA (one-way) followed by Tukey´s post hoc test.
References
Albert, I., Böhm, H., Albert, M., Feiler, C. E., Imkampe, J., Wallmeroth, N., et al. (2015). An RLP23–SOBIR1–BAK1 complex mediates NLP-triggered immunity. Nat. Plants. 1, 15140. doi: 10.1038/nplants.2015.140
Albert, I., Hua, C., Nürnberger, T., Pruitt, R. N., Zhang, L. (2020). Surface sensor systems in plant immunity. Plant Physiol. 182, 1582–1596. doi: 10.1104/pp.19.01299
Arenas, Y. C., Kalkman, E. R. I. C., Schouten, A., Dieho, M., Vredenbregt, P., Uwumukiza, B., et al. (2010). Functional analysis and mode of action of phytotoxic Nep1-like proteins of Botrytis cinerea. Physiol. Mol. Plant Pathol. 74, 376–386. doi: 10.1016/j.pmpp.2010.06.003
Asai, S., Shirasu, K. (2015). Plant cells under siege: plant immune system versus pathogen effectors. Curr. Opin. Plant Biol. 28, 1–8. doi: 10.1016/j.pbi.2015.08.008
Bi, K., Liang, Y., Mengiste, T., Sharon, A. (2022). Killing softly: a roadmap of Botrytis cinerea pathogenicity. Trends. Plant Sci. 28 (2), 211–222. doi: 10.1016/j.tplants.2022.08.024
Bi, K., Scalschi, L., Jaiswal, N., Mengiste, T., Fried, R., Sanz, A. B., et al. (2021). The Botrytis cinerea Crh1 transglycosylase is a cytoplasmic effector triggering plant cell death and defense response. Nat. Commun. 12, 2166. doi: 10.1038/s41467-021-22436-1
Boutrot, F., Zipfel, C. (2017). Function, discovery, and exploitation of plant pattern recognition receptors for broad-spectrum disease resistance. Annu. Rev. Phytopathol. 55, 257–286. doi: 10.1146/annurev-phyto-080614-120106
Brito, N., Espino, J. J., González, C. (2006). The endo-β-1,4-xylanase xyn11A is required for virulence in Botrytis cinerea. Mol. Plant Microbe Interact. 19, 25–32. doi: 10.1094/MPMI-19-0025
Choquer, M., Rascle, C., Gonçalves, I. R., de Vallée, A., Ribot, C., Loisel, E., et al. (2021). The infection cushion of Botrytis cinerea: a fungal 'weapon' of plant-biomass destruction. Environ. Microbiol. 23, 2293–2314. doi: 10.1111/1462-2920.15416
Cui, H., Tsuda, K., Parker, J. E. (2015). Effector-triggered immunity: from pathogen perception to robust defense. Annu. Rev. Plant Biol. 66, 487–511. doi: 10.1146/annurev-arplant-050213-040012
Dagvadorj, B., Outram, M. A., Williams, S. J., Solomon, P. S. (2022). The necrotrophic effector ToxA from parastagonospora nodorum interacts with wheat NHL proteins to facilitate Tsn1-mediated necrosis. Plant J. 110, 407–418. doi: 10.1111/tpj.15677
Dean, R., Van Kan, J. A., Pretorius, Z. A., Hammond-Kosack, K. E., Di Pietro, A., Spanu, P. D., et al. (2012). The top 10 fungal pathogens in molecular plant pathology. Mol. Plant Pathol. 13, 414–430. doi: 10.1111/j.1364-3703.2011.00783.x
Denton-Giles, M., McCarthy, H., Sehrish, T., Dijkwel, Y., Mesarich, C. H., Bradshaw, R. E., et al. (2020). Conservation and expansion of a necrosis-inducing small secreted protein family from host-variable phytopathogens of the sclerotiniaceae. Mol. Plant Pathol. 21, 512–526. doi: 10.1111/mpp.12913
Doehlemann, G., Ökmen, B., Zhu, W., Sharon, A. (2017). Plant pathogenic fungi. Microbiol. Spectr. 5 (1), 703–726. doi: 10.1128/9781555819583.ch34
Eizner, E., Ronen, M., Gur, Y., Gavish, A., Zhu, W., Sharon, A. (2017). Characterization of botrytis-plant interactions using PathTrack©: an automated system for dynamic analysis of disease development. Mol. Plant Pathol. 18, 503–512. doi: 10.1111/mpp.12410
Franco-Orozco, B., Berepiki, A., Ruiz, O., Gamble, L., Griffe, L. L., Wang, S., et al. (2017). A new proteinaceous pathogen-associated molecular pattern (PAMP) identified in ascomycete fungi induces cell death in solanaceae. New Phytol. 214, 1657–1672. doi: 10.1111/nph.14542
Frías, M., González, C., Brito, N. (2011). BcSpl1, a cerato-platanin family protein, contributes to Botrytis cinerea virulence and elicits the hypersensitive response in the host. New Phytol. 192, 483–495. doi: 10.1111/j.1469-8137.2011.03802.x
Frías, M., González, M., González, C., Brito, N. (2016). BcIEB1, a Botrytis cinerea secreted protein, elicits a defense response in plants. Plant Sci. 250, 115–124. doi: 10.1016/j.plantsci.2016.06.009
Gao, Y., Faris, J. D., Liu, Z., Kim, Y. M., Syme, R. A., Oliver, R. P., et al. (2015). Identification and characterization of the SnTox6-Snn6 interaction in the Parastagonospora nodorum-wheat pathosystem. Mol. Plant Microbe Interact. 28, 615–625. doi: 10.1094/MPMI-12-14-0396-R
Gui, Y. J., Chen, J. Y., Zhang, D. D., Li, N. Y., Li, T. G., Zhang, W. Q., et al. (2017). Verticillium dahliae Manipulates plant immunity by glycoside hydrolase 12 proteins in conjunction with carbohydrate-binding module 1. Environ. Microbiol. 19, 1914–1932. doi: 10.1111/1462-2920.13695
Heard, S., Brown, N. A., Hammond-Kosack, K. (2015). An interspecies comparative analysis of the predicted secretomes of the necrotrophic plant pathogens Sclerotinia sclerotiorum and Botrytis cinerea. PloS One 10, e0130534. doi: 10.1371/journal.pone.0130534
Jeblick, T., Leisen, T., Steidele, C. E., Albert, I., Müller, J., Kaiser, S., et al. (2023). Botrytis hypersensitive response inducing protein 1 triggers noncanonical PTI to induce plant cell death. Plant Physiol. 191, 125–141. doi: 10.1093/plphys/kiac476
Jones, J. D. G., Dangl, J. L. (2006). The plant immune system. Nature 444, 323–329. doi: 10.1038/nature05286
Kettles, G. J., Bayon, C., Canning, G., Rudd, J. J., Kanyuka, K. (2017). Apoplastic recognition of multiple candidate effectors from the wheat pathogen Zymoseptoria tritici in the nonhost plant Nicotiana benthamiana. New Phytol. 213, 338–350. doi: 10.1111/nph.14215
Kim, K.-T., Jeon, J., Choi, J., Cheong, K., Song, H., Choi, G., et al. (2016). Kingdom-wide analysis of fungal small secreted proteins (SSPs) reveals their potential role in host association. Front. Plant Sci. 7, 186. doi: 10.3389/fpls.2016.00186
Leisen, T., Werner, J., Pattar, P., Safari, N., Ymeri, E., Sommer, F., et al. (2022). Multiple knockout mutants reveal a high redundancy of phytotoxic compounds contributing to necrotrophic pathogenesis of Botrytis cinerea. PloS Pathog. 18, e1010367. doi: 10.1371/journal.ppat.1010367
Liebrand, T. W., van den Berg, G. C., Zhang, Z., Smit, P., Cordewener, J. H., America, A. H., et al. (2013). Receptor-like kinase SOBIR1/EVR interacts with receptor-like proteins in plant immunity against fungal infection. Proc. Natl. Acad. Sci. U.S.A. 110, 10010–10015. doi: 10.1073/pnas.1220015110
Lo Presti, L., Lanver, D., Schweizer, G., Tanaka, S., Liang, L., Tollot, M., et al. (2015). Fungal effectors and plant susceptibility. Annu. Rev. Plant Biol. 66, 513–545. doi: 10.1146/annurev-arplant-043014-114623
Lorang, J., Kidarsa, T., Bradford, C. S., Gilbert, B., Curtis, M., Tzeng, S. C., et al. (2012). Tricking the guard: exploiting plant defense for disease susceptibility. Science 338, 659–662. doi: 10.1126/science.1226743
Lorang, J. M., Sweat, T. A., Wolpert, T. J. (2007). Plant disease susceptibility conferred by a “resistance”. gene. Proc. Natl. Acad. Sci. U.S.A. 104, 14861–14866. doi: 10.1073/pnas.0702572104
Ma, L., Salas, O., Bowler, K., Oren-Young, L., Bar-Peled, M., Sharon, A. (2017). Genetic alteration of UDP-rhamnose metabolism in Botrytis cinerea leads to the accumulation of UDP-KDG that adversely affects development and pathogenicity. Mol. Plant Pathol. 18, 263–275. doi: 10.1111/mpp.12398
Ma, Z., Song, T., Zhu, L., Ye, W., Wang, Y., Shao, Y., et al. (2015). A Phytophthora sojae glycoside hydrolase 12 protein is a major virulence factor during soybean infection and is recognized as a PAMP. Plant Cell. 27, 2057–2072. doi: 10.1105/tpc.15.00390
Mousavi-Derazmahalleh, M., Chang, S., Thomas, G., Derbyshire, M., Bayer, P. E., Edwards, D., et al. (2019). Prediction of pathogenicity genes involved in adaptation to a lupin host in the fungal pathogens Botrytis cinerea and Sclerotinia sclerotiorum via comparative genomics. BMC Genomics 20, 385. doi: 10.1186/s12864-019-5774-2
Oliver, R. P., Friesen, T. L., Faris, J. D., Solomon, P. S. (2012). Stagonospora nodorum: From pathology to genomics and host resistance. Annu. Rev. Phytopathol. 50, 23–43. doi: 10.1146/annurev-phyto-081211-173019
Oome, S., Raaymakers, T. M., Cabral, A., Samwel, S., Böhm, H., Albert, I., et al. (2014). Nep1-like proteins from three kingdoms of life act as a microbe-associated molecular pattern in Arabidopsis. Proc. Natl. Acad. Sci. U.S.A. 111, 16955–16960. doi: 10.1073/pnas.1410031111
Postma, J., Liebrand, T. W. H., Bi, G., Evrard, A., Bye, R. R., Mbengue, M., et al. (2016). Avr4 promotes cf-4 receptor-like protein association with the BAK1/SERK3 receptor-like kinase to initiate receptor endocytosis and plant immunity. New Phytol. 210, 627–642. doi: 10.1111/nph.13802
Reboledo, G., Agorio, A., Vignale, L., Batista-García, R. A., Ponce De León, I. (2021). Botrytis cinerea Transcriptome during the infection process of the bryophyte Physcomitrium patens and angiosperms. J. Fungi. 7, 11. doi: 10.3390/jof7010011
Schellenberger, R., Touchard, M., Clément, C., Baillieul, F., Cordelier, S., Crouzet, J., et al. (2019). Apoplastic invasion patterns triggering plant immunity: plasma membrane sensing at the frontline. Mol. Plant Pathol. 20, 1602–1616. doi: 10.1111/mpp.12857
Shao, D., Smith, D. L., Kabbage, M., Roth, M. G. (2021). Effectors of plant necrotrophic fungi. Front. Plant Sci. 12, 687713. doi: 10.3389/fpls.2021.687713
Shi, G., Friesen, T. L., Saini, J., Xu, S. S., Rasmussen, J. B., Faris, J. D. (2015). The wheat Snn7 gene confers susceptibility on recognition of the Parastagonospora nodorum necrotrophic effector SnTox7. Plant Genome. 8, 1–10. doi: 10.3835/plantgenome2015.02.0007
Shlezinger, N., Minz, A., Gur, Y., Hatam, I., Dagdas, Y. F., Talbot, N. J., et al. (2011). Anti-apoptotic machinery protects the necrotrophic fungus Botrytis cinerea from host-induced apoptotic-like cell death during plant infection. PloS Pathog. 7, e1002185. doi: 10.1371/journal.ppat.1002185
Stergiopoulos, I., de Wit, P. J. G. M. (2009). Fungal effector proteins. Annu. Rev. Phytopathol. 47, 233–263. doi: 10.1146/annurev.phyto.112408.132637
Valero-Jiménez, C. A., Veloso, J., Staats, M., van Kan, J. A. L. (2019). Comparative genomics of plant pathogenic Botrytis species with distinct host specificity. BMC Genomics 20, 203. doi: 10.1186/s12864-019-5580-x
van der Burgh, A. M., Postma, J., Robatzek, S., Joosten, M. H. A. J. (2019). Kinase activity of SOBIR1 and BAK1 is required for immune signalling. Mol. Plant Pathol. 20, 410–422. doi: 10.1111/mpp.12767
van Ooijen, G., van den Burg, H. A., Cornelissen, B. J., Takken, F. L. (2007). Structure and function of resistance proteins in solanaceous plants. Annu. Rev. Phytopathol. 45, 43–72. doi: 10.1146/annurev.phyto.45.062806.094430
Veloso, J., van Kan, J. A. L. (2018). Many shades of grey in botrytis-host plant interactions. Trends Plant Sci. 23, 613–622. doi: 10.1016/j.tplants.2018.03.016
Zhang, L., Kars, I., Essenstam, B., Liebrand, T. W. H., Wagemakers, L., Elberse, J., et al. (2014). Fungal endopolygalacturonases are recognized as microbe-associated molecular patterns by the Arabidopsis receptor-like protein RESPONSIVENESS TO BOTRYTIS POLYGALACTURONASES1. Plant Physiol. 164, 352–364. doi: 10.1104/pp.113.230698
Zhang, M. Z., Sun, C. H., Liu, Y., Feng, H. Q., Chang, H. W., Cao, S. N., et al. (2020). Transcriptome analysis and functional validation reveal a novel gene, BcCGF1, that enhances fungal virulence by promoting infection-related development and host penetration. Mol. Plant Pathol. 21, 834–853. doi: 10.1111/mpp.12934
Zhou, J. M., Zhang, Y. (2020). Plant immunity: danger perception and signaling. Cell 181, 978–989. doi: 10.1016/j.cell.2020.04.028
Zhu, W., Ronen, M., Gur, Y., Minz-Dub, A., Masrati, G., Ben-Tal, N., et al. (2017). BcXYG1, a secreted xyloglucanase from Botrytis cinerea, triggers both cell death and plant immune responses. Plant Physiol. 175, 438–456. doi: 10.1104/pp.17.00375
Keywords: CDIPs, Botrytis cinerea, BcCDI1, elicitor, plant defense
Citation: Zhu W, Dong H, Xu R, You J, Yan D-z, Xiong C, Wu J and Bi K (2023) Botrytis cinerea BcCDI1 protein triggers both plant cell death and immune response. Front. Plant Sci. 14:1136463. doi: 10.3389/fpls.2023.1136463
Received: 03 January 2023; Accepted: 04 April 2023;
Published: 25 April 2023.
Edited by:
Mario Serrano, Center for Genomic Sciences, National Autonomous University of Mexico, MexicoReviewed by:
Kei Hiruma, The University of Tokyo, JapanJan A. L. Van Kan, Wageningen University and Research, Netherlands
Copyright © 2023 Zhu, Dong, Xu, You, Yan, Xiong, Wu and Bi. This is an open-access article distributed under the terms of the Creative Commons Attribution License (CC BY). The use, distribution or reproduction in other forums is permitted, provided the original author(s) and the copyright owner(s) are credited and that the original publication in this journal is cited, in accordance with accepted academic practice. No use, distribution or reproduction is permitted which does not comply with these terms.
*Correspondence: Kai Bi, kaibi@whpu.edu.cn; Ran Xu, rxu@whpu.edu.cn; Jingmao You, jingmaoyou@126.com